Breast cancer imaging with magnetic resonance imaging: Approaches and pulse sequences
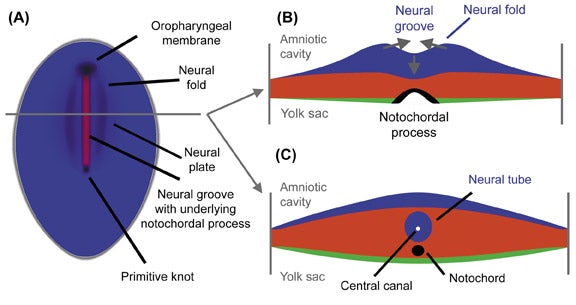
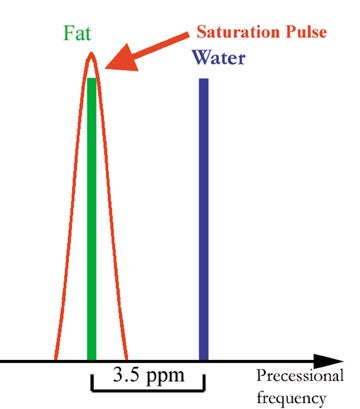
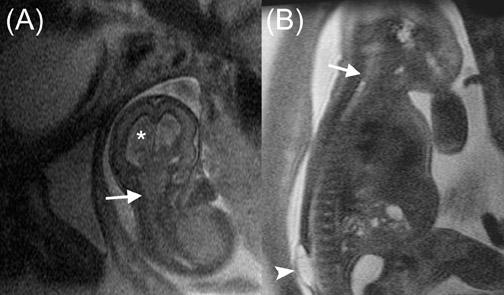
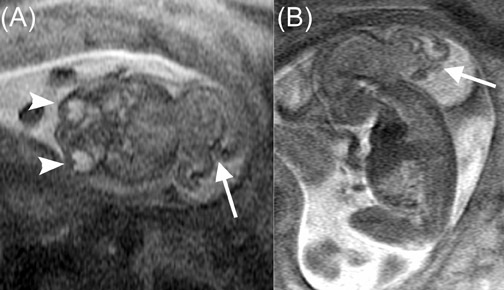
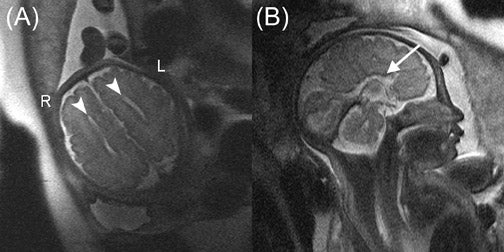
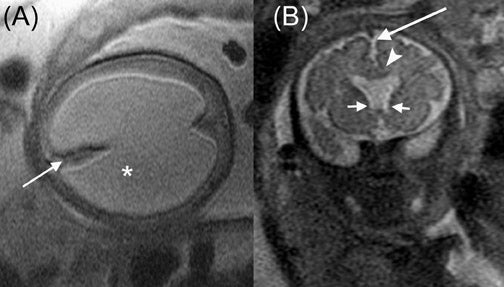
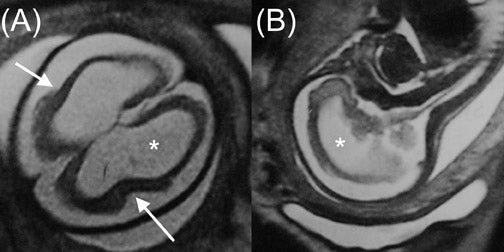
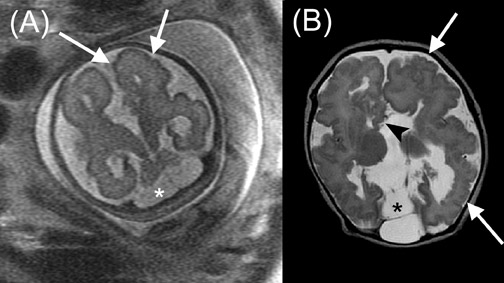
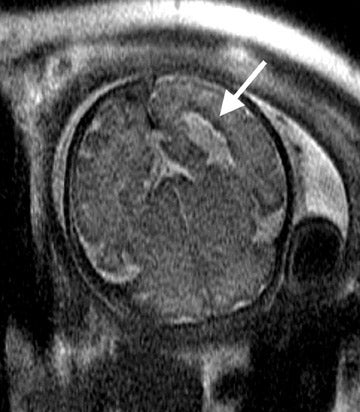
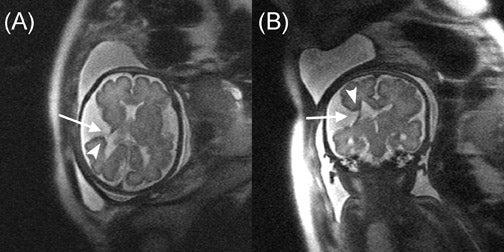
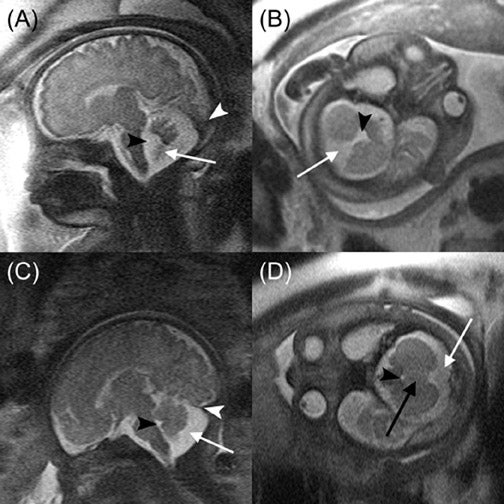
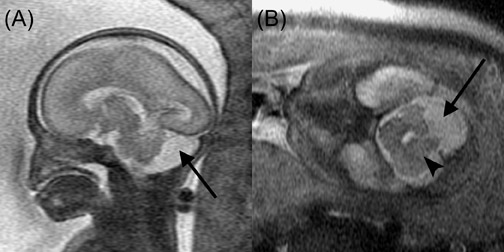
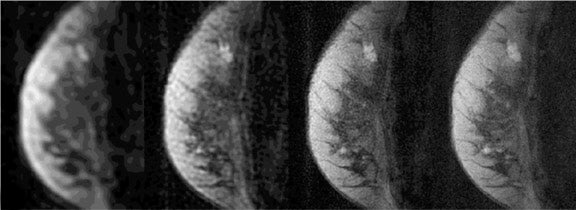
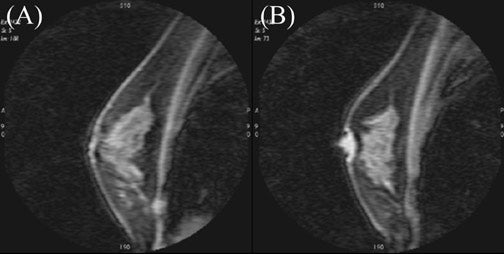
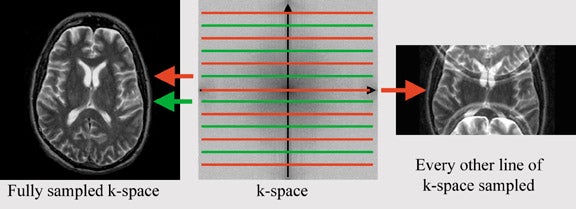
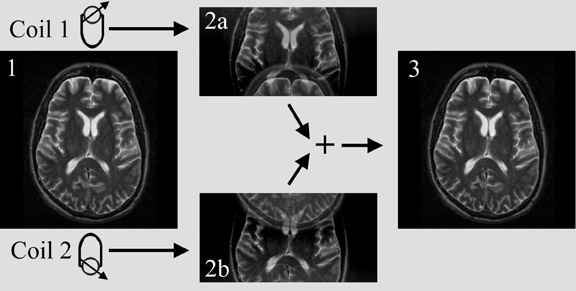
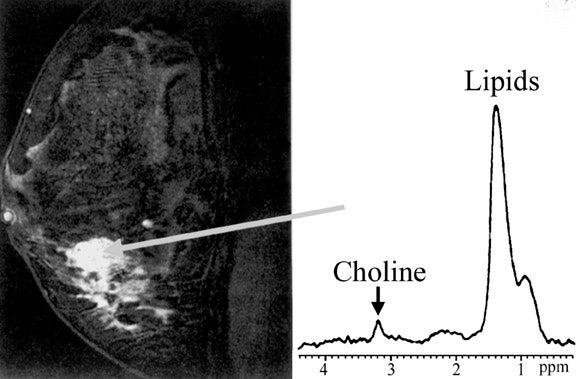
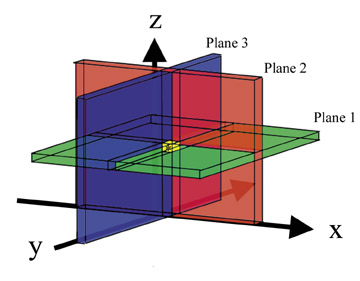
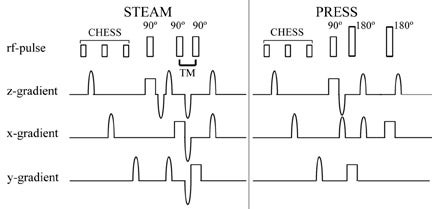
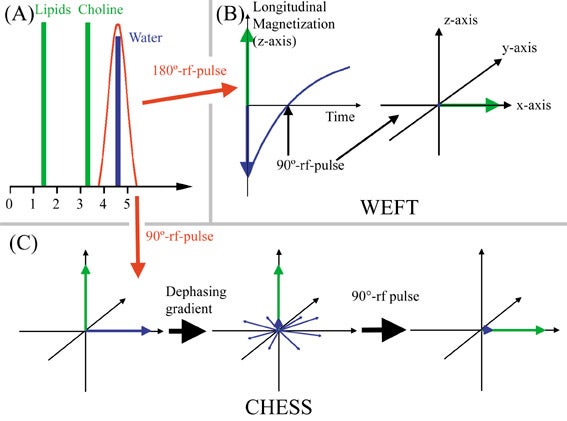
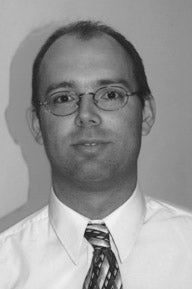
Dr. Rassner is currently a 3rd-year Resident in Diagnostic Radiology at the University of Utah, Salt Lake City, UT. He received his MD from Eberhard-Karls University, Tübingen, Germany.
Breast cancer is a disease of high prevalence and mortality. It is estimated that >211,300 women in the United States alone were newly diagnosed with invasive breast cancer and >39,800 women died of their disease in 2003, 1 making breast cancer the second leading cause, after lung cancer, of malignancy-related death in women. Many factors influence patient survival, a number of which cannot be changed, such as race and age at diagnosis, while others can be affected. Because early detection of breast cancer greatly improves the chances for successful treatment and survival, it must be physicians' foremost goal to diagnose breast cancer at the earliest stage possible. 2 These efforts must be balanced against the psychological and financial burden that diagnostic measures could entail.
The leading imaging modality for breast cancer screening and diagnosis is mammography. While the sensitivity of mammography is reported to be 70% to 90%, the specificity is much lower. Mammography is currently the primary imaging modality used to decide which lesions require biopsy. The current recommended positive predictive value for biopsies is 25% to 40%. 3-5 Fewer unnecessary biopsies are desirable for many reasons, including economic factors and patient care aspects.
Magnetic resonance imaging (MRI) of the breast has proved to be the most sensitive method of detecting implant rupture in the augmented breast. In contrast, the use of MRI for the detection of breast cancer is considerably less straightforward. Although it offers the promise of a noninvasive problem-solving tool with a mild increase in sensitivity and a substantial increase in specificity, there is currently no consensus on MRI standards regarding pulse sequences or interpretation criteria. Therefore, many different imaging and interpretation approaches exist, leading to a wide range of reported sensitivities and specificities. 6,7 While certain pulse sequences are designed to maximize spatial resolution, others aim to detect dynamic enhancement characteristics with high temporal resolution, at the cost of spatial resolution. Newer approaches aim to obtain images with both high spatial and temporal resolution. MR spectroscopy can detect choline compounds, a known sign of malignancy.
This article will provide an overview of current and investigational MRI approaches for detection of malignant breast masses. This discussion presumes a basic understanding of MRI physics. Readers desiring a review of basic imaging physics are referred to any of several standard texts. 8
General considerations
Because there is no universally accepted standard for MRI of the breast, many studies employ different parameters regarding field strength, coil configuration, pulse sequence choices (ie, two-dimensional versus three-dimensional acquisition, temporal versus spatial resolution, pulse sequences contain- ed in the MR breast examination, etc.). However, no matter which protocol is followed, high signal-to-noise ratio (SNR) and good spatial resolution are desirable. The coil should be as close to the target organ as possible to fulfill these demands. Different varieties of breast coils exist. The most commonly used are double breast coils, on which the patient rests in the prone position (Figure 1). Breast coil design variations include open or closed design, compression or no compression, and number of channels per breast. Multiple channels are required to perform parallel imaging.
Most imaging protocols employ intravenous contrast. Unlike mammography, where lesion detectibility is increased in the fatty breast, fat may obscure an enhancing lesion in MRI, as it may become isointense to fat after gadolinium administration. Thus, most breast imaging protocols utilize some form of fat suppression.
Fat suppression strategies for breast magnetic resonance imaging
Several fat-suppression methods exist. A commonly used method is called fat saturation or chemical saturation and employs a radiofrequency (RF) pulse to nullify fat signal. 9,10 Fat signal suppression with this technique relies on the fact that fat and water have different precession frequencies, called Larmor frequencies. The majority of the signal in nonfatty tissues comes from water protons. Although the magnetic field of clinical MR scanners is very homogenous, it is modulated by different tissues to differing degrees. This effect is called chemical shift and refers to the difference in magnetic field strength that the protons of fat and nonfatty tissues experience. The resulting difference in Larmor frequency of fat and water is 3.5 ppm (approximately 220 Hz on a 1.5T scanner). Radiofrequency pulses can be made more or less frequency-specific, meaning that they contain a wider or narrower range of frequencies. A RF pulse with a wider range of frequency will affect water and fat protons, while a RF pulse with a narrow range of frequencies may only affect the fat protons. The fat spins are turned into the transverse plane and are dephased by application of a gradient, thus destroying the fat signal (Figure 2).
A second commonly used approach is subtraction of precontrast images from postcontrast images acquired with the same imaging parameters. 11,12 This technique requires minimal patient motion and matching of precontrast with postcontrast images. Many other fat suppression techniques have been utilized, described elsewhere. 13-16
High spatial resolution magnetic resonance imaging
Architectural features of a lesion, such as shape, margins, and density, and of the surrounding parenchyma, such as architectural distortion, play an important role in the interpretation of mammograms. It follows intuitively to utilize some of these criteria with breast MRI. While some of these criteria are easily transferred to breast MRI, such as margins; others are unique to standard mammography, such as lesion density. Breast MRI offers additional diagnostic criteria, such as the presence of septations, T1 and T2 signal characteristics, pattern, and degree of enhancement. Different interpretation models have been proposed, taking these features into consideration 15,17-19 (Figures 3 and 4).
Breast MRI for evaluation of architectural features requires high spatial resolution. The most commonly used approach is a spoiled gradient-recalled echo (SPGR) sequence before and after contrast application. 17,20,21 This pulse sequence can be used with or without fat-suppression techniques. Gradient-recalled echo sequences differ from standard spin-echo sequences in two ways: First, instead of a 90º RF pulse, a RF-pulse with a smaller flip angle is applied. Secondly, there is no 180º refocusing pulse. This permits imaging with very short repetition times (TR) and therefore fast imaging with high resolution for the following reasons:
If a RF-pulse causes a smaller flip angle (the angle that is formed between the magnetic vector before and after the RF pulse is applied), a part of the magnetic vector is in the transverse plane, while a portion of longitudinal magnetization remains. The transverse component of the magnetic vector can be measured, while the significant residual longitudinal magnetization allows for faster recovery of the full longitudinal magnetization (Figure 5). Therefore TR can be shortened. In standard spin-echo sequences, the presence of a refocusing pulse puts limitations on the minimum time after which the signal can be acquired (time of echo [TE]). This is due to the fact that the pulse itself takes some time, and the resulting echo should not overlap with the refocusing pulse to prevent zipper artifacts. Thus, the absence of a refocusing pulse allows a much shorter TE (Figure 6).
Contrast-enhanced sequences are typically T1-weighted (some
newer approaches differ from that and are mentioned later). While
in spin-echo sequences, the T1- or T2-weighting is determined by TE
and TR alone, additional factors are important in gradient-
recalled imaging, such as the flip angle and spoiling versus
refocusing. Gradient-recalled echo sequences show T2*-properties
rather than T2-properties. T2*is shorter than T2 due to additional
dephasing by a variety of factors, including field inhomogeneities,
and chemical shift. A difference in longitudinal magnetization must
exist when the next RF pulse
is applied to obtain T1-weighted se-quences. If the flip angle is
small, the residual magnetization after the RF pulse is only
marginally smaller than fully relaxed longitudinal magnetization.
Even differences in T1-property will not lead to a significant
difference in longitudinal magnetization and thus transverse
magnetization and signal after the RF pulse has been applied
(Figure 7A). If the flip angle is increased, the longitudinal
magnetization after the RF pulse is notably smaller than before the
pulse. Thus, differences in T1-property will lead to differences in
longitudinal magnetization and therefore transverse magnetization
and signal, after the RF pulse has been applied (Figure 7B). Also,
a certain time is required for the differences in longitudinal
magnetization to develop. Therefore the TE needs to be relatively
longer than that for T2*-weighted gradient-echo sequences.
8
Spoiling refers to dephasing of residual transverse magnetization
after the signal has been acquired. Because the TR in
gradient-recalled sequences is relatively short, residual
magnetization is present in the transverse plane by the time the
next RF pulse has to be applied. Because T2*-properties determine
the rate of transverse magnetization decay, differences of residual
transverse magnetization between different tissues are caused by
T2*. If the RF pulse is applied, before the signal is dephased, the
new transverse magnetization will add to the residual transverse
magnetization. This can be desirable when T2*-weighted images are
acquired, but is undesirable for T1-weighted images. To avoid the
interference between T2* and T1, the residual transverse
magnetization is dephased (spoiled) either via a gradient or by
applying successive RF-pulse with a phase offset in respect to each
other. The latter is called RF-spoiling and is used in most modern
MR scanners (Figure 6).
The earlier mentioned advantages of gradient-recalled echo sequences are achieved at the expense of much greater sensitivity to susceptibility artifact. Also, if the echo time is chosen inappropriately in sequences without fat suppression, the spins of water and fat can possess opposite phase. This leads to reduced or nulled signal of voxels containing fat and water, resulting in an etching artifact.
For earlier mentioned reasons, gradient-recalled sequences can be acquired rapidly, which means that images with a large image matrix can be obtained within short enough times, to sample different time points after contrast enhancement.
High temporal resolution
magnetic resonance imaging
Solid tumors consist of three basic components: the cancer cells themselves; blood vessels, accounting for approximately 1% to 10% of tumor volume in tumors large enough to have their own blood supply; and a collagen-rich matrix, the interstitium. 22 Many malignant tumors release a variety of cytokines and growth factors, such as vascular endothelial growth factor (VEGF). These induce growth of new vessels from already existing microvessels. These vessels are abnormal in structure and function, 23 exhibiting increased vessel density and permeability. In addition, they exhibit an increased amount of arteriovenous shunts. Although many details have been observed regarding tumor morphologic characteristics, the exact mechanism of altered contrast dynamics remain unclear. It has been shown that many breast tumors show an earlier onset of enhancement and a washout effect, which the majority of benign breast masses do not exhibit. The commonly used classification scheme distinguishes types 1a and 1b, type 2, and type 3 time courses. Type 1a is continuous enhancement throughout the entire acquisition. Type 1b indicates slowing down of enhancement in the late postcontrast phase. A lesion classified as type 2 shows a plateau after the initial enhancement, while a type 3 lesion shows loss of signal after the initial enhancement due to washout. 12 Type 1 lesions are benign in 94% of the cases, while type 3 lesions are malignant in 87% of the cases (Figure 8). 12 Type 2 lesions fall in between; however, they are more likely malignant than benign. 12 In addition, the enhancement rates are also used by some for characterization of a suspicious lesion. Again, different protocols are used by different groups, and the reported sensitivities and specificities to diagnose invasive cancer vary from 76% to 91% and 67% to 96%, respectively. 12,21,24
The majority of MRI protocols employing high temporal resolution fall into two categories: T1-weighted SPGR with regular readout 12,24,25 or echoplanar imaging (EPI) readout. 26,27
The regular SPGR sequences are identical to the earlier mentioned sequence (in the section on high spatial resolution imaging), except for one key difference: to reduce the duration of image acquisition, the image matrix is reduced. The number of phase-encoding steps has a dominant effect on imaging time, while the number of frequency-encoding steps minimally influences imaging time. The desired resolution in phase-encoding direction equals the number of phase-encoding steps needed in standard sequences. For every phase-encoding step, the pulse sequence has to be repeated, while varying the phase-encoding gradient. Each measurement corresponds to one line in k-space. K-space is the data space that contains spatial frequency information (Figure 9). The center line in k-space represents zero-phase shift, thus signal acquisition in the absence of a phase-encoding gradient. Fourier transformation converts this data space into image space (the MR image). If resolution in phase encoding direction is reduced by 50%, imaging time will also be reduced by 50%, allowing for faster acquisition and, therefore, higher temporal resolution.
Echoplanar imaging refers to a technique with which multiple lines in k-space--multiple measurement--can be acquired rapidly within one pulse sequence. While in regular gradient-echo and spin-echo sequences the pulse sequence has to be repeated for every line of k-space, while varying the phase- encoding gradient, in EPI, the entire k-space can be acquired within one pulse sequence. The maximum negative phase- encoding gradient is applied (corresponding to the bottom line in k-space) and a measurement acquired to allow aquisition of all of k-space. Immediately after the measurement, a positive phase-encoding gradient is applied, slightly reducing the negative phase shift. Another measurement is acquired in the presence of a phase-encoding gradient (Figure 10). The short application of a positive phase-encoding gradient and measurement is repeated until the maximum positive phase shift is reached (corresponding to the top-most line in k-space). In this manner, a rapid acquisition of an entire slice is possible. The earlier described technique is referred to as blipped EPI (short blips of the phase-encoding gradient). For reasons that go beyond the scope of this review, it can be desirable to acquire k-space with several acquisitions, which is referred to as multishot EPI. In multishot EPI, the individual acquisitions are interleaved. The overall contrast of the EPI sequence depends on the root sequence (the sequence before the echoplanar readout--such as a T2-weighted spin-echo or a T1-weighted gradient-echo). Many other EPI-techniques are available with different types of k-space sampling strategies.
Combining speed and resolution
The benefits of high temporal resolution and of high spatial resolution studies have been shown earlier. It is easy to understand that, ideally, one would want an imaging sequence that combines high temporal resolution with high spatial resolution to use morphologic as well as dynamic enhancement to characterize lesions. While the benefits of combining two different sets of diagnostic criteria is intuitive, it has also been shown that higher spatial resolution allows for better definition of dynamic contrast enhancement characteristics. 28 The reason is that smaller lesions can be volume-averaged, with tissues exhibiting benign enhancement characteristics. The benign tissue can then mask the smaller focus of malignant enhancement pattern. Different approaches are used, which so far have not been very commonly utilized, but will likely be more frequently seen in the future. One approach samples the center of k-space more frequently than the periphery, 29,30 the second approach utilizes parallel imaging approaches. 31,32
K-space (or data space) differs in several respects from the image space. While in the regular image the position along the x- and y-axis refers to spatial location, in k-space every point contains information about the entire image (spatial frequency information). One of the consequences of this is that the center of k-space contains information regarding predominantly the overall image contrast, while the periphery of k-space contains information about interfaces. Thus, after enhancement, the center of k-space will contain most of the information regarding changes in signal due to enhancement. The periphery of k-space changes little after contrast application, because the interfaces between structures do not change. If the periphery of k-space can be sampled less frequently than the center of k-space, the imaging time is shorter, because fewer lines in k-space are sampled. Saranathan et al 30 sampled the entire k-space with every fourth acquisition. Outer k-space data from the full data set are then combined with each of the previous fractional data sets to generate four full-resolution images at high temporal resolution (Figure 11).
Song et al 29 report a different technique utilizing earlier described properties of k-space. K-space can be sampled in a radial fashion (Figure 12). The more lines that are acquired, the higher the resolution will be. Lower resolution images can be sampled more rapidly (fewer lines in k-space) and, therefore, more frequently. If the radial lines between each acquisition are slightly rotated in respect to the prior acquisition, multiple acquisitions can be combined to form a single image with high spatial and low temporal resolution (Figure 12). If the individual acquisitions are Fourier-transformed, images with low spatial, but high temporal, resolution are obtained. The individual acquisitions can thus be combined in different ways to form images of varying temporal and spatial resolution (Figure 13).
Another innovative approach is using parallel imaging techniques, such as sensitivity encoding (SENSE) (Figure 14), simultaneous acquisition of spatial harmonics (SMASH), or variations of these techniques. 31-34 The concept of parallel imaging described here illustrates SENSE imaging. If k-space is undersampled (for instance only every other line in k-space is acquired), a reduced field of view and aliasing, also called wrap-around artifact, will occur (Figure 15). Obviously, the imaging time will be reduced if k-space is undersampled. In parallel imaging, a multiple-element coil is used. The signal is acquired by each element separately. Each coil element has a certain sensitivity profile. This means that spins closer to the coil element will create a stronger signal than the same spins at a greater distance from this coil. Two coil elements obtain different measurements of the same object due to their different location and a particular sensitivity profile. Thus, spatial information is encoded in the signal by the coil sensitivity. If the sensitivity profile of the coil elements is known, this information can be used to combine the measurements of the individual coil elements and unwrap the image, resulting in an image with the full field of view and no aliasing (Figure 16). The parallel imaging approach can be combined with any pulse sequence. The possible acceleration factor depends on the number of coil elements and receiver channels. Parallel imaging can be used either to acquire an image faster, or with higher resolution or larger field of view within the same scan time.
Magnetic resonance spectroscopy for breast mass characterization
Imaging of the breast for breast cancer detection aims to fulfill two major goals: high sensitivity for detection of breast lesions and reliable differentiation of benign from malignant lesions (specificity). MR spectroscopy offers an adjunctive tool for lesion characterization utilizing other characteristics (choline content) than earlier mentioned techniques. Single-voxel proton-spectroscopy is most widely used and, although not a tool for screening an entire breast, it can play a role in improving specificity (Figure 17). 35 In addition, proton MR spectroscopy may be a useful tool to evaluate response to neoadjuvant chemotherapy. 36 Phosphorus 31 spectroscopy has also been used in evaluation of breast cancer. 37 However, the signal is lower, because there is less 31 P in the breast than protons, and special hardware is required. A recent review of several MR spectroscopy studies showed an average sensitivity and specificity of 92%. 38 In a subgroup of patients (¾40 years of age) the sensitivity and specificity approached 100%. It has been shown that there are large differences in the metabolite composition of breast tumors and surrounding normal breast tissue. 39 Choline is the most widely used marker for malignancy of breast lesions and has been shown to be a valid marker in vivo and ex vivo. 38,40 In fine-needle biopsy specimens, the sensitivity and specificity for distinguishing benign lesions from invasive cancer with proton MR spectroscopy was 95% and 96%, respectively. 40 Choline is commonly viewed as a marker of membrane turnover; however, it has been shown that some breast cancer cell lines exhibit a in-creased expression of choline kinase, leading to increased choline/phosphocholine levels. 41 This technique has certain limitations: Choline is detectable in a majority of breast-feeding women without the presence of a malignant lesion, 42 and ductal carcinoma in situ shows only a mild elevation of choline ex vivo 40 and is unreliably or not diagnosed with in vivo MR-spectroscopy. 43,44
The most commonly used single-voxel MR spectroscopy sequences are point-resolved spectroscopy (PRESS) 42-44 and stimulated acquisition mode (STEAM). 35,36 Both sequences use 3 RF pulses that are made slice-selective in 3 orthogonal planes. Only the spins experiencing all 3 pulses will have a spin-echo at the time of measurement. In this way, a target volume is selected (Figure 18). STEAM uses three 90º pulses. The time between the second and third pulse is called mixing time (TM). During TM, the transverse magnetization created by the first 90º RF pulse is oriented antiparallel to the z-axis and, therefore, decays with T1-relaxation. Because T1 is longer than T2, less magnetization decays (Figure 19). Despite this, STEAM has about half the signal of PRESS, which uses one 90º RF pulse and two 180º pulses. 45 Different TEs (31 to 450 msec) are used, with the longer TEs commonly being a multiple of 135 msec. TE is chosen this way to allow the highest sensitivity for detection of lactate. Lactate has a double peak at slightly different resonant frequencies. The individual components of the doublet peak show an in- and out-of-phase phenomenon in respect to each other with a frequency of approximately 7 Hz. Thus, lactate will be in-phase every 135 msec (1Ž7 sec). This is due to j-coupling effects. Lactate is not routinely used as a criterion in evaluation of breast lesions, although it has been shown to be elevated in breast cancer cell lines. 46 The standard MR spectroscopy sequences are set up in such a fashion to allow detection of lactate.
Suppression of water signal is vital for proton MR spectroscopy to detect small metabolite quantities. Water-elimination Fourier-transform technique (WEFT) and chemical shift selective saturation (CHESS) are the most commonly used techniques. WEFT is a frequency-selective inversion recovery sequence. A 180º inversion pulse affecting only the water spins is applied, aligning the water spins antiparallel to the main magnetic field (antiparallel to the longitudinal axis). T1-relaxation of water then takes place. When the longitudinal magnetization of water traverses zero, the regular 90º RF pulse is applied. CHESS utilizes a water-selective 90º RF pulse, flipping only the water spins into the transverse plane. The water spins in the transverse plane are then dephased in the transverse plane (Figure 20). This is often repeated with dephasing gradients applied along different planes, before the regular 90º RF pulse is applied. In both techniques water will not have greatly reduced longitudinal magnetization at the time the regular 90º RF pulse is applied and will, therefore, yield little signal.
Other techniques
A variety of other MR techniques have been applied to breast cancer evaluation, but are not currently widely used and, therefore, are not discussed here. Such techniques include MR elastography, 47,48 MR perfusion scanning, 49,50 diffusion-weighted imaging, 51,52 and steady-state gradient-recalled echo with balanced gradients (FIESTA, TRUE-FISP). 53,54 Some of these techniques also require additional hardware or certain system performance characteristics. MRI of breast cancer is not limited to evaluation of the primary breast lesion. Different techniques have been used for staging and evaluation of nodal disease, including utilization of ultrasmall superparamagnetic iron oxide particles. 55,56
Conclusion
Breast MR imaging has the potential to become a standard for breast cancer evaluation. It offers high sensitivity and specificity. However, at this point, there is no consensus on an imaging standard for MRI of the breast and many different approaches are utilized: Evaluation of morphologic features, contrast dynamics, and choline content of the lesion are most commonly used. The combination of these techniques holds the most promise to offer the highest sensitivity and specificity for breast mass evaluation, supplementing the established techniques of mammography and ultrasound.