3T MRI in clinical practice
Images
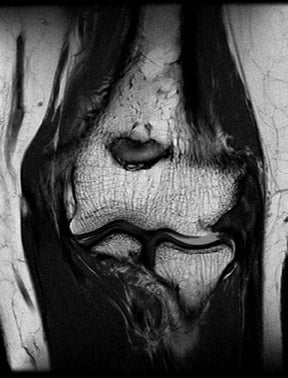
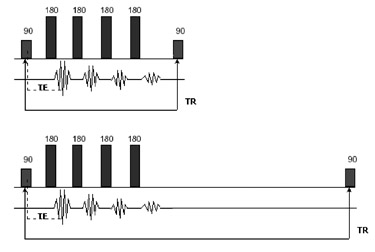
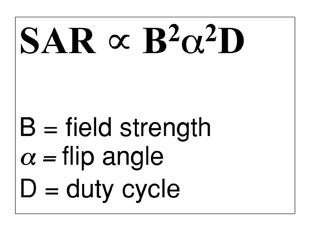
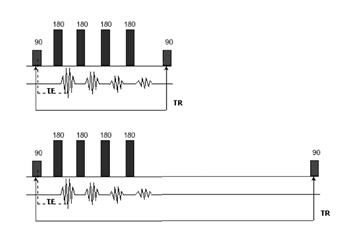
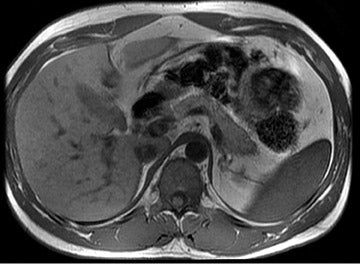
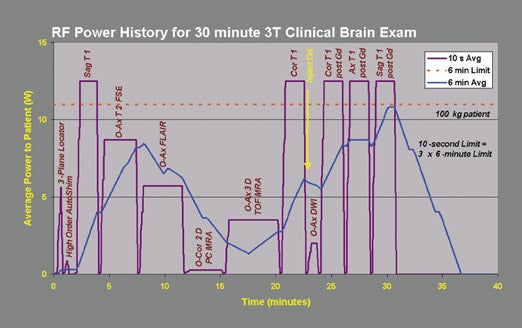
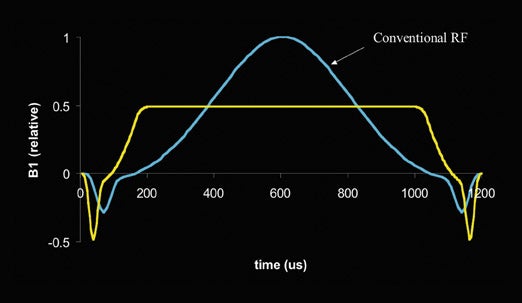
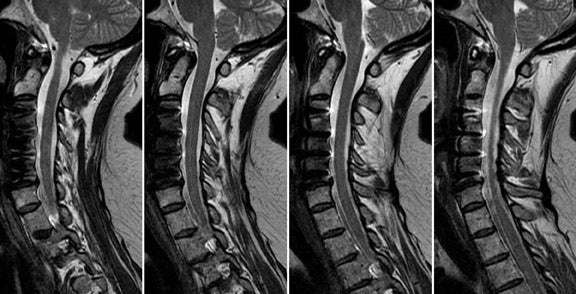
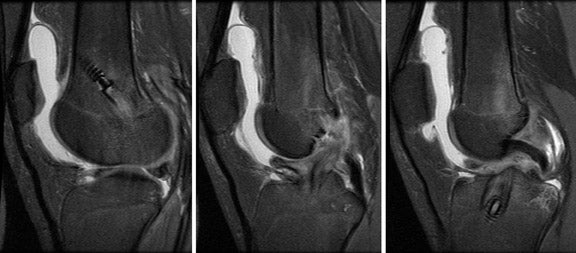
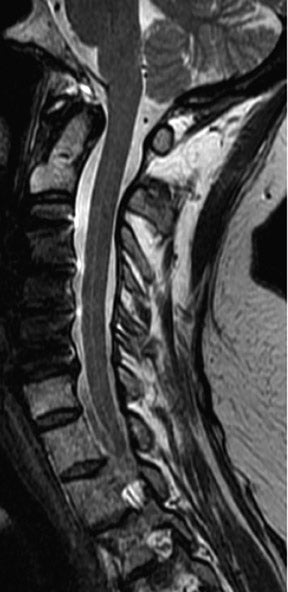
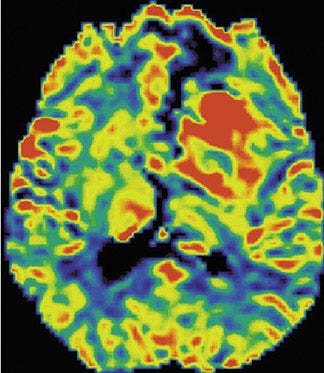
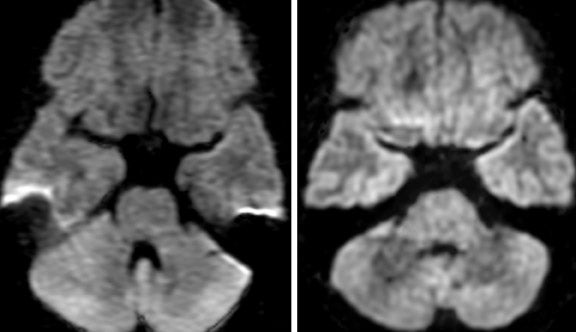
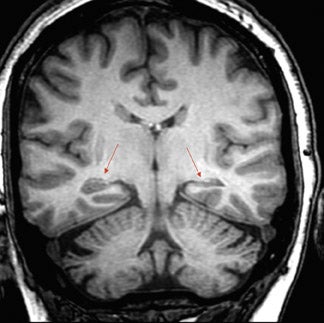
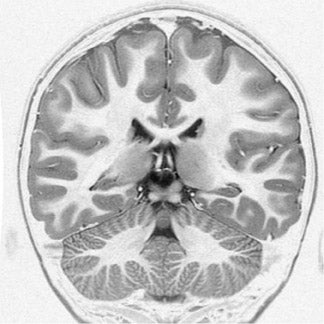
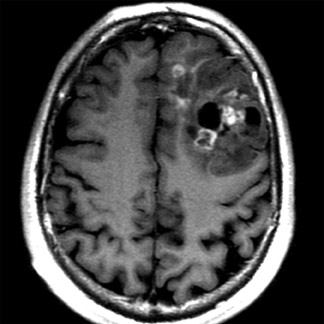
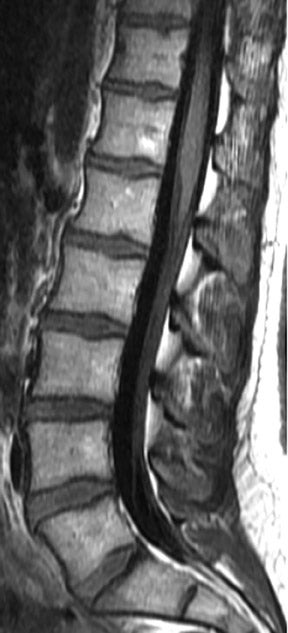
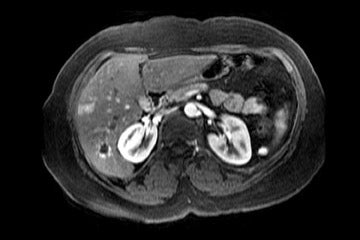
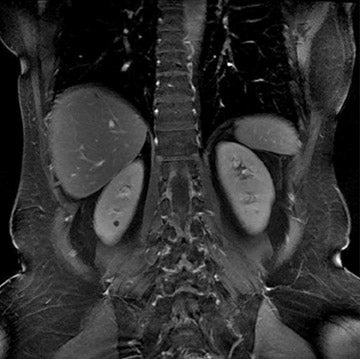
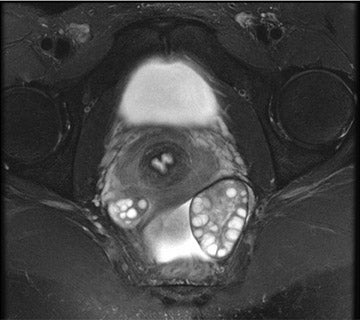
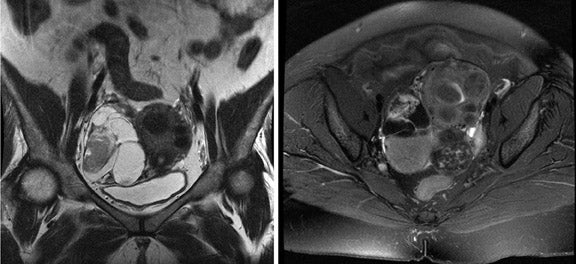
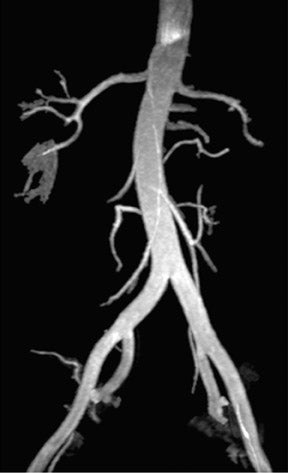
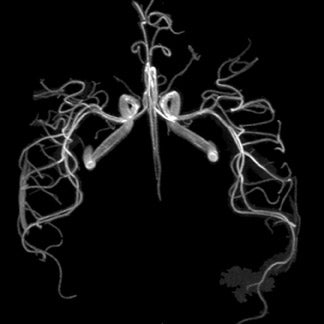
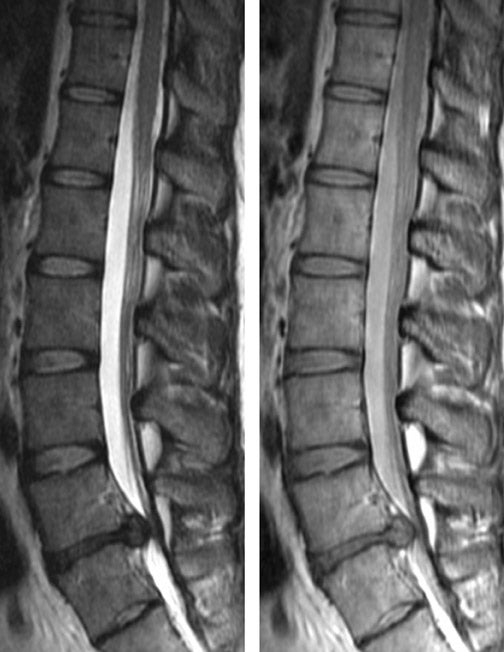
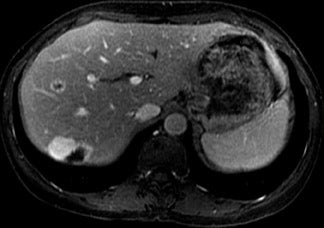
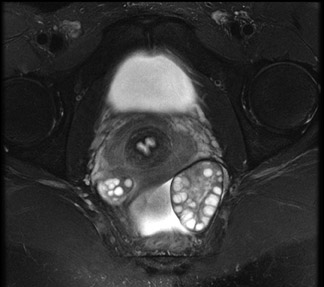
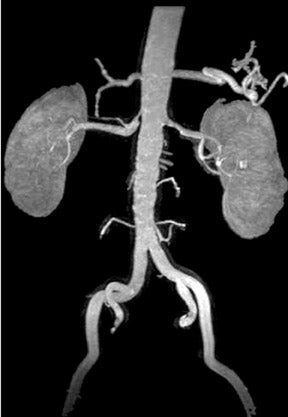
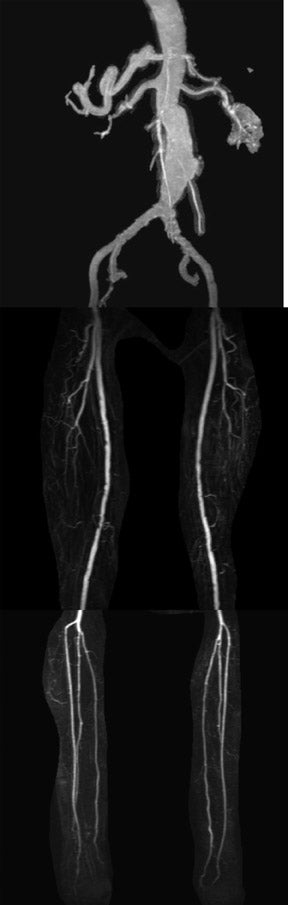
Over the last several years, systems operating at higher fields have become more prevalent, particularly at research centers. An informal survey of the market reveals that approximately 250 whole-body-capable MR systems are currently in operation with the majority of recent installations in the clinical practice setting. Market data in late 2004 indicate that 3T systems make up 25% of new high-field MR purchases.
Fueling the shift in interest from 1.5 to 3T from primarily a research device to clinical practice validates that what was once considered very high-field MR (3T) is practical, feasible, and, indeed, currently or potentially superior to 1.5T for clinical indications throughout the body. The driving forces behind this increased penetration of 3T scanners into the clinical setting include reduced concerns over surface coil availability, radiofrequency (RF) deposition limit, higher ambient noise, system homogeneity, increased magnetic susceptibility and chemical shift effects, and reduced tissue contrast. Also, this shift results from the documentation of incremental benefits of 3T over 1.5T with respect to image quality and efficiency.
Specific absorption rate
Specific absorption rate (SAR) is a measure of energy deposited by an RF field in a given mass of tissue. SAR is established by the International Electrotechnical Commission (IEC) to not exceed 8 watts per kg (W/kg) of tissue for any 5-minute period or 4 W/kg for a whole body averaged over 15 minutes. 1 Dissipation of RF energy in the body can result in tissue heating. The doubling of field from 1.5T to 3T leads to a quadrupling of SAR (Figure 1). Therefore, SAR considerations effectively limit scanner performance.
Manipulations traditionally used to limit SAR include reducing acquisition flip angle (eg, from 180˚ on fast spin-echo [FSE] and ~40˚ on gradient-recalled echo [GRE]), which could potentially affect image contrast. Longer echo time acquisitions commonly used with high-per-formance gradient systems and fat-suppression techniques commonly used with musculoskeletal imaging exacerbate the duty cycle load. Reducing duty cycle by using a longer repetition time (TR) than the minimum necessary (building in cooling time, in a sense) is an effective technique but comes at the expense of somewhat longer scan times (Figure 2). Parallel imaging is another powerful method of reducing RF exposure as well as scan times by reducing the number of phase-encoding steps that are performed. The typical trade-off in signal-to-noise ratio (SNR) (a parallel imaging factor of 2 reduces SNR by 40%) is balanced by improved surface coil design and the higher signal acquired at 3T. Another technique of managing SAR concerns is to interleave SAR-intensive sequences with low RF deposition scans, eg, follow a fat-suppressed FSE scan with a gradient-echo acquisition before starting the next FSE scan.
Innovative methods of reducing SAR without image compromise are, or will soon be, available. New magnet designs now seen in the clinical setting are inherently more SAR-efficient than were earlier generation systems. Clever pulse sequence manipulations such as applying magnetization transfer prepulses only at the center one third of k-space can maintain improved tissue contrast while depositing considerably less RF energy. Advances in pulse sequence design such as reshaping RF and gradient waveforms reduces peak RF power up to 40% compared with conventional techniques. The development and availability of more local transmit/receive surface coils will also reduce SAR deposition and further enhance efficiency.
Ambient noise
Sound pressure levels (SPLs) increase with field strength. The noise levels at 3T approach twice that of 1.5T and can be in excess of 130 dBA 2 (the IEC and the U.S. Food and Drug Administration limit permissible sound levels to 99 dBA). Higher gradient performance comes at the cost of higher SPL as well. Magnet length also influences the gradient noise generated, thus the shorter bore systems sold today are inherently louder.
Methods of reducing SPL include passive approaches, such as the routine use of earplugs, as well as active noise cancellation via headphones. Reduced gradient performance for certain applications is another approach, but by nature, this limits clinical efficacy. Some currently available 3T systems are equipped with advances, such as acoustically shielded vacuum-based bore liners that keep noise levels below certain limits while maintaining full gradient performance.
Tissue contrast issues
T1 relaxation times are prolonged at 3T with respect to 1.5T leading to reduced contrast resolution on traditional (short TR, short echo time [TE]) spin-echo (SE) acquisitions. These considerations do not plague other methods for obtaining T1 contrast, such as RF-spoiled gra-dient-recalled, magnetization prepared techniques such as inversion recovery (IR), or magnetization transfer (MT) 3-dimensional spoiled gra-dient-recalled (SPGR) (Figures 3 and 4). Inversion recovery techniques that produce superior T1 contrast at 1.5T, such as phase-sensitive IR for the brain (Figure 5) and T1 fluid attenuated inversion recovery (FLAIR) for the brain, spine, and musculoskeletal system, are equally well suited to higher field imaging and can yield spectacular results. With parallel imaging techniques, T1 studies are faster and higher in resolution than those obtained at 1.5T. A routine shift to high bandwidth, to a moderate echo-train (ET)-FSE (T1 FLAIR) from spin-echo, has the additional benefit of reducing susceptibility artifact, which is a benefit in patients who have had surgery or who have metal implants, and chemical shift effect sensitivity as well.
While the relaxivity of gadolinium is not significantly different at 1.5T from at 3T, the longer T1 of tissues at 3T contributes to an increase in conspicuity of enhancement (greater contrast-to-background ratio). Therefore, many sites utilize a lower dose of contrast (0.05 mmol/kg) for routine brain imaging purposes. T2 values in biological tissues are unchanged or only slightly decreased with increases in field strength. T2* effects scale with field strength, and 3T studies are thus more sensitive to deposition of blood products and tissue mineralization (Figures 6 and 7). Conversely, susceptibility artifacts are proportionally more problematic at 3T (Figures 8 and 9). The higher SNR afforded by 3T augmented by the power of the latest generation phased-array coils allows a variety of techniques to compensate for T2* effects, including the use of parallel imaging and higher bandwidth with longer ET-FSE acquisitions.
Diffusion imaging
The greater signal intensity afforded at 3T is particularly enticing for diffusion-weighted imaging (DWI) needs. Signal-to-noise ratio can be marginal for routine clinical imaging purposes at 1.5T, and the quest for higher B values (>1000 s/mm 2 ), thinner slices (<3 mm), and white matter anisotropy mapping (tensor imaging) further stresses the SNR equation (Figure 10). Diffusion-weighted imaging studies at high field are typically acquired using echoplanar imaging (EPI) techniques. These single-shot studies are inherently prone to susceptibility artifact, which can limit evaluation of structures in close proximity to the bony skull base and air-filled paranasal sinuses. Since susceptibility effects scale with field strength, these artifacts are proportionally worse at higher field. Parallel imaging techniques are routinely applied on modern 3T systems equipped with optimized surface coils and broadband reconstruction hardware, effectively balancing these considerations by decreasing the echo-spacing (ES) and TE of the scan. This reduces susceptibility artifact and ameliorates signal loss due to T2 decay on these long ET acquisitions (Figure 11). When widely available, less artifact-prone FSE techniques (eg, Propeller) to obtain DWI should become popular at 3T (Figure 12).
BOLD studies
Perhaps the greatest neuroimaging impact of 3T is in enhancing the capability of functional MR imaging (fMRI). The greater susceptibility, contrast sensitivity, and higher SNR inherent to 3T scanning can produce up to a 40% increase in detected activation with blood-oxygenation-level-dependent (BOLD) imaging over 1.5T. 3 Improved contrast resolution enhances the success rate of these procedures for routine presurgical mapping of eloquent cortex (eg, sensorimotor, language) and coupled with scanner integrated paradigm delivery may lead to utilization in community practice to evaluate disorders such as dementia and psychiatric disorders (Figure 13).
Time-of-flight neuro MR angiography
The longer T1 of background tissues can be exploited for superior inflow MR angiography (TOF MRA). Scanning techniques employ lower flip angles that reduce SAR deposition as well as pulsation artifacts. The higher SNR provided by 3T with 8-channel surface coils encourages routine utilization of high imaging matrices (512 × 1024) producing studies that can rival the resolution of digital subtraction angiography (DSA) (Figures 14 and 15). Optimized coils coupled with parallel imaging techniques maintain scan times similar to or shorter than those at 1.5T.
MR spectroscopy
Chemical shift doubles when moving from 1.5T to 3T, resulting in improved spectral resolution allowing evaluation of metabolites that may be obscured at 1.5T. This factor along with the higher SNR of 3T may increase the efficacy of proton and multinuclear spectroscopy of many disorders (Figure 16).
Body imaging
Specific absorption rate considerations reduce slices available per given time, encouraging multiple breath-hold acquisitions. Motion-resist-ant techniques with single-shot FSE and respiratory-triggered multishot FSE are also commonly utilized. Eight-channel phased-array surface coil designs optimized for parallel imaging ameliorate many SAR-based limitations. Studies of the abdomen and pelvis are routinely accomplished with thinner slices and higher imaging matrices, comparable to those utilized with computed tomography, facilitating comparison and lesion characterization (Figures 17 and 18). The higher SNR afforded by 3T may also facilitate applications such as spectroscopy and might obviate the need for endocavitary surface coils for advanced applications, such as prostate imaging (Figures 19 and 20).
Body vascular
The higher SNR of 3T coupled with parallel imaging compatible surface coils produce high-quality, perhaps higher resolution, contrast-enhanced vascular studies with greater consistency than at 1.5T. Lowering the flip angle reduces SAR, and thus acquisition time. The longer T1 values of background tissues serves to augment visualization of intravascular contrast, potentially allowing a reduction in contrast dose administered (Figure 21). While full-body vascular coils are yet to become available, the increasing importance of multistation time-resolved MRA techniques at the expense of so-called bolus-chasing reduces their significance (Figure 22).
Musculoskeletal imaging
Spine
Eight-channel phased-array coils are widely available for spine imaging. Practical considerations yield studies that are generally higher in resolution and somewhat faster than at 1.5T (Figures 23 and 24). While susceptibility is a theoretical concern, long ET high-bandwidth acquisitions yield excellent image quality even for patients with implanted metal hardware.
Joint imaging
Responsible for upwards of 20% of the study volume of the typical clinical scanner, the quality of joint imaging is a major factor in determining the financial feasibility of higher field MR. Until recently, coil availability has been limited and SAR concerns are prominent as high duty cycle applications, such as fat suppression and long ET FSE, are common. The homogeneity of the latest generation short-bore devices is critical as joints are rarely scanned near isocenter and fat suppression is so important for adequate contrast resolution.
Fortunately, high-quality, high SNR, often phased-array surface coils are becoming available and are generally providing studies that are recognizably superior to those from 1.5T systems in somewhat less time (Figure 25). Offering higher spatial resolution, 3T MR may yield additional use in the study of smaller parts and cartilage than do examinations obtained at 1.5T (Figure 26). Studies obtained with older and less sophisticated coils (eg, quadrature) benefit from the SNR of 3T to be competitive with studies obtained with more advanced surface coils at 1.5T (Figure 27).
Receive-only coils require SAR-intensive body coil transmission that limits performance. The future availability of transmit/receive capable surface coils should significantly augment efficiency and further extend quality.
The higher SNR of 3T allows utilization of higher resolution protocols with smaller fields-of-view (FOV), thinner slices, and larger imaging matrices. The greater susceptibility sensitivity of 3T should make tissue mineralization easier to appreciate. Instrumented joints can be imaged with manageable artifact with high bandwidth, long ET-FSE, and T1-weighted IR-FSE techniques.
Conclusion
Fundamentally, 3T MR imaging offers twice the signal of 1.5T. Specific absorption rate considerations are becoming less of a limitation due to technical advances and surface coils that are available for all core applications. The greater amount of signal can be manipulated to make scanning more comfortable (scan times that may be half as long) or more sensitive (higher resolution) to smaller lesions (eg, small and early multiple sclerosis plaques). Typical utilization combines both benefits in a given scan. The greater sensitivity to magnetic susceptibility effects offers unique benefits in functional neuroimaging as well as improving sensitivity to brain hemorrhage. Practical considerations make studies of the brain, spine, chest, abdomen, pelvis, as well as the vasculature and extremities obtained at 3T consistently higher in quality than those obtained at 1.5T.