Perspective on dose reduction: What are we measuring and why?
Images
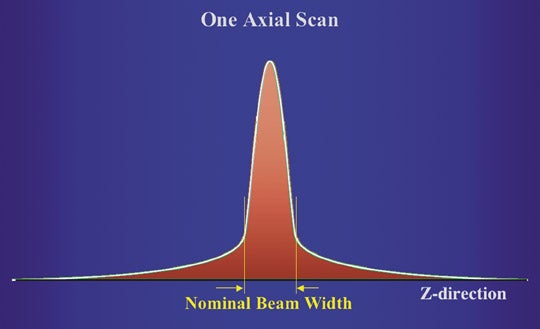
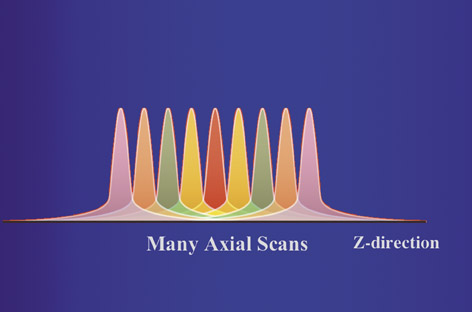
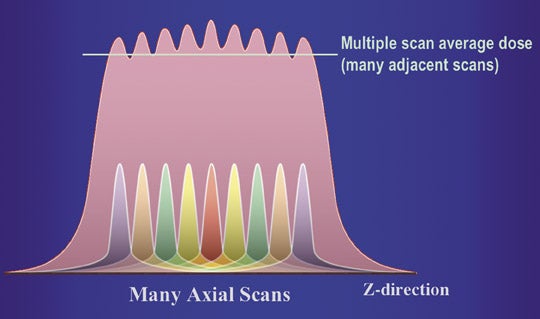
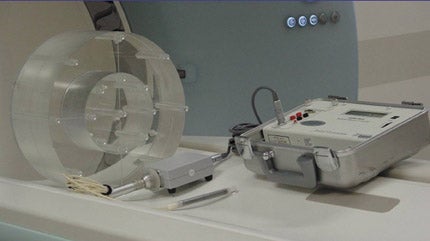
Dr. McCollough, is a Professor of Radiologic Physics and Director, CT Clinical Innovation Center, Department of Radiology, Mayo Clinic, Rochester, MN.
Dr. McCollough discloses that she has received research support from the National Institutes of Health, the Society of Gastrointestinal Radiologists and Siemens Healthcare. She is also the recipient of the Mayo Novel Methodology Development Award.
In a discussion of computed tomography (CT) dose reduction, we need to first understand what we are measuring. Specifically in terms of radiation, it is necessary to understand how we have arrived at our current measurement strategies, how we assess risk of radiation-induced cancer and how we convey that information to patients in a meaningful way. For the exposure from a single gantry rotation, the dose distribution along the Z axis is not rectangular. Rather, the dose distribution has a “tail” that extends beyond the nominal beam width (Figure 1A). At the axis of rotation, which is also known as the gantry isocenter, the nominal width of the radiation dose distribution should match the width of the detector array capturing the transmitted X-ray information.
For most clinical exams, data is acquired from multiple gantry rotations, typically as the patient is translated through the gantry. This results in a set of overlapping radiation dose distributions along the Z axis (Figure 1B). The highest dose level in each curve is referred to as the peak radiation dose. However, in a study comprised of multiple scans at different Z axis positions, the tails of each dose distribution add up to a level that is higher than the peak radiation dose from a single scan. With enough scans, the average dose level will reach an equilibrium value, which is referred to as the multiple-scan average dose (MSAD) (Figure 1C).
In the early days of CT, directly measuring MSAD was a cumbersome process. Physicists manually packed many thermoluminescent dosimeters (TLDs) into an acrylic holder, which was then placed inside an acrylic phantom and scanned using a clinical protocol. The TLDs in the holders were then manually unloaded and placed into a machine to read out the radiation dose values. This labor-intensive process had to be repeated for any scan protocol of interest. The other issue that made this process prohibitively time consuming was the limit on X-ray power. To make measurements for multiple scan protocols, the X-ray tubes would get very hot and the physicist would have to wait a considerable amount of time between subsequent exposures.
The roots of CTDI
In 1981, Shope et al. devised a more practical method to describe the dose delivered by a CT scan.1They estimated the equilibrium dose,or the MSAD, using a single gantry rotation. This concept became known as the “CT Dose Index” (CTDI). The CTDI integrates all the radiation delivered by a single scan (one gantry rotation) and normalizes it to the nominal beam width. This normalization builds in a way to assess dose deficiency. For example, comparing two different scanners, one having a dose distribution that is as wide as the nominal beam width and the other having a dose distribution that is twice as wide as the nominal beam width, the scanner with the wider beam will deliver twice as much radiation from the single scan. However, the integrated dose is normalized to the nominal beam width (10 mm) in both cases, so that the CTDI of the scanner with the wider beam is twice as large as the CTDI of the scanner with the more-narrow beam. This directly indicates that the scanner where the width of the actual dose distribution matches the nominal beam width is twice as dose efficient as the other scanner, where the actual beam width is twice as wide.
Shope and colleagues demonstrated that the CTDI provides a good estimate of the MSAD when the table increment between scans is equal to the nominal beam width. However, there are some caveats about using CTDI to estimate MSAD. First, the scan protocol must contain enough scans so that the MSAD has reached an equilibrium value. Second, the ion chamber or other dosimeter used in the CTDI measurement must be long enough to measure all the tails of the dose distribution. Pragmatically, the phantoms used for these measurements are shorter than the average patient’s torso (Figure 2), and so they do not produce as much scattered radiation. This means that CTDI measurements in the typical CTDI phantoms underestimate the extent of the tails that are actually produced in adult patients. This implies that the CTDI will underestimate the average dose (MSAD) that would occur in patients. This effect can be as high as 30%.
The CTDI is, however, very easy to measure and allows the physicist to take dose measurements for a wide range of scan parameters. Another strength of CTDI is that it is an extremely well-defined measurement that is used internationally by standards organizations, regulators and professional societies. It is absolutely essential, however, to realize that CTDI is not a measurement of dose to any given patient. Rather, it is a standardized way to quantitate the radiation output of a CT system.
While CTDI can be measured at the edge or the center of a phantom, a weighted CTDI (CTDIw) is more often quoted in order to use a single value to represent the scanner output. The weighted CTDI combines one third of the CTDI at the gantry isocenter with two thirds of the CTDI at the phantom periphery.2,3 For the head CTDI phantom, which has a diameter of 16 cm, the CTDI values at the center and edge are typically the same. However in the body CTDI phantom, which is 32 cm in diameter, the CTDI in the center is about one half of the value of the CTDI at the edge.
From CTDIw to CTDIvol
Whether we talk about the edge, center, or weighted CTDI, the principle is the same: The integral under the radiation-dose profile is normalized to the Z-axis width, over which data is collected. However, it is essential to take into account the Z-axis spacing between consecutive scans. For example, two different exams could have the same value of CTDIw, but for an exam with a pitch of 2, the patient table moves through the gantry twice as fast as for a pitch of 1. Thus, the pitch-2 exam would deliver half the dose to the patient compared with the pitch-1 exam. The volume CTDI (CTDIvol) was adopted to better estimate the MSAD, taking into account gaps or overlaps between consecutive scans.
CTDIvol(estimate of MSAD) = CTDIw(measurements from one axial scan)/Pitch
Pitch = Table Increment per rotation/Total nominal scan width
On modern scanners, the CTDIvolis reported on the scanner console prior to the initiation of any scan. It is a relative reflection of the radiation output of the scanner that allows users to verify proper operation of the scanner. Actual dose to a given patient, however, depends very much on the size and shape of the patient. Thus, CTDIvol, which is measured using standardized cylindrical acrylic phantoms, allows us to compare the dose efficiency between different scan protocols or scanners, but should not be used to represent the dose to a given patient.
Uses of CTDIvol
Because of the standardization of CTDIvol, it can be used to confirm that a system is operating according to manufacturers’ specifications, and it allows users to compare a readily verifiable dose value between different systems and protocols. Without such standardization, users could not be sure that one scanner performs as well as another. Just as car manufacturers must report fuel consumption understandardized testing conditions to inform consumers regarding fuel efficiency (e.g. miles per gallon), CTDI tells us about the dose efficiency of a CT scanner.
CTDIvolalso allows operators to adjust scanner output according to a specific patient’s size or the exam type/diagnostic task. Professional organizations can survey the typical CTDIvol values used for standard-sized adults (or children) for a specific exam (for example, CT colography or CT angiography), and these data can be used to help users to prescribe scanner settings that result in similar patient doses relative to other practices. The technologist can monitor the CTDIvol during the exam, which helps them to flag errors in scan prescription. For example, in an average-size patient where an imaging center typically uses 18 mGy for a routine abdomen exam, prior to starting the scan, the technologist can quickly check if the prescribed scan has a CTDIvol of approximately 18 mGy. If a parameter was entered incorrectly, resulting in a CTDIvol of 3 mGy or 30 mGy (again for an average-sized patient), the technologist has immediate feedback alerting them to recheck the scan prescription. For larger patients, the CTDIvol should, of course, increase and for smaller patients, it should decrease. These “patient-size dose adjustments” follow a predictable scale. Thus, knowledge of a reasonable CTDIvol value for a standard patient, combined with a size-based technique chart, will ensure proper dose selection across all patient sizes.
The consistency provided by measuring scanner output with a standardized phantom set and technique is invaluable in comparing dose data across a range of scanner technologies and exam protocols. I am able to review CTDIvol data from as far back as 1980, and compare those numbers with what we use in our practice today, allowing me, for example, to document the factor of 2 to 3 reduction in dose that has occurred since the early 1980s for a routine abdomen CT exam.
Risk
While CTDIvol gives us a common language with which to understand how much radiation a scanner is producing, it does not measure doses within a patient, such as to a specific organ, because any single dosimetry phantom, or even a set of phantoms, could not possibly compensate for all the variations in patient size and shape. CTDIvol can be used to reconstruct the dose distribution in a given patient, based on the size and shape information contained in the CT images, and this allows medical physicists to obtain reasonable estimates of organ doses.
But why are organ doses necessary? The reason we want to know organ doses is to estimate and communicate risk information. A patient will not benefit from knowing that the CTDIvol of his or her exam was 1 mGy vs. 10 mGy vs. 100 mGy unless they have a frame of reference upon which to gauge the possible health effects associated with a given amount of radiation.
Our largest source of information about the effects of whole-body irradiation comes from atomic bomb survivors, whose health has been monitored since 1945 by the Radiation Effects and Research Foundation. Summaries of the current consensus understanding of radiation risk are given in a series of reports known as the BEIR reports. These reports are authored by the National Research CouncilCommittee on Biological Effects of Ionizing Radiation (BEIR), and can be used to estimate risks based on patient gender and age at the time of the exposure and the dose to a variety of radiation-sensitive organs.
Effective dose
Effective dose (E), is a mathematical construct that takes into account the dose to specific organs and the radiation sensitivity of those organs. It allows us to estimate the risks from a CT exam, for example of the chest, which irradiates only a part of the torso, from what we know about the bomb survivors, who received exposures to their entire body. Effective dose was initially developed for radiation protection purposes, and so uses averages over both genders and all ages. Its weakness is that it does not take into account the potential benefit from a medical radiation exposure nor the age and gender of any given patient. It is, however, now widely used to estimate risk from medical exposures, even though the patient receiving the exam may not live long enough to ever develop a potential cancer due to their medical condition. In such a case, the risk of the CT scan would be zero.
To estimate effective dose, mathematical models of patients having various degrees of realism are combined with the information about the radiation exposure delivered by the scanner (from CTDIvol). The gold standard method uses Monte Carlo calculations (i.e. statistical modelling) to estimate organ doses from a particular scan protocol on a given scanner. Because scanner technology is constantly changing, various methods are used to take into account the particular characteristics of a given CT system. These calculations yield a list of organ dose estimates, which are then multiplied by radiation sensitivity weighting factors. These tissue-sensitivity weighting factors are specified by the International Council on Radiation Protection (ICRP) in their various reports. To date, 3 publications have addressed this topic, ICRP Reports 26, 60 and 103. The weighting factors for a given organ have been modified over time as the data from the atomic bomb survivors and other exposed cohorts are reassessed. Thus, even for identical organ-dose estimates, the calculated value of E can differ based on which ICRP report was used.
A convenient shortcut to estimating effective dose uses the dose-length product (DLP), which is calculated by multiplying the CTDIvol by the length of the CT scan. The DLP is then multiplied by one of 5 generic values that are based only on body regions (head, neck,head and neck, chest, or abdomen and pelvis). These values have similarly been revised over time, with the most recent values being published in 2006.4,5
A recent paper by Martin5provides an enlightening discussion on the uncertainties associated with estimates of effective dose, and should be read and understood by every physician, physicist or administrator wishing to use effective dose for a specific patient or to predict excess cancer risks. Martin establishes that there is an uncertainty in estimated values of E from a medical exposure on the order of +/- 40%, even when the same patient model is used. Uncertainties in the estimation of cancer risk, however, compound these uncertainties such that estimated risk for a specific model of a patient (a reference patient) can fluctuate by a factor of +/-3. Because individuals can vary considerably from a reference patient, estimation of cancer risk for an individual has uncertainties of a factor of +/- 5 associated with them.
Dose reference levels
An alternative method of reporting and managing patient dose is through dose reference levels (DRLs). They were first mentioned by the ICRP in Report 60 and later recommended in greater detail in Report 73. They are a form of investigation level that can be used as a simple test to identify situations where patient dose is unusually high. If consistently exceeded, a local review of procedures and equipment should be performed to determine whether the exam has been adequately optimized. If it has not been optimized, dose-reduction measures are encouraged. However, DRLs are supplements to professional judgment and do not provide a dividing line between “good” and “bad” medicine.Additionally, they apply only to medical exposures, not to occupational and public exposures. They have no link to dose limits or constraints and they are the result of generic exam optimization.
In practice, DRLs are typically set around the 75th percentile of the observed distribution of doses for average-sized patients. This information allows physicians to know how their protocols stack up against regional, national, or international practices. Specific DRLs are selected by professional medical bodies and reviewed at appropriate intervals. The values are typically specific to a given country orregion.
In the United Kingdom, DRLs have been used for several years and researchers have shown declines in mean dose as a result. There was a 30% decline in mean dose between 1984 and 1995 and 50% decline in mean dose between 1985 and 2000. In order to achieve greater clinical adoption, DRLs must be defined in terms of an easily and reproducibly measured dose metric. They also must use technique parameters that reflect those used in a CT department’s routine clinical practice, and surveys of technique parameters must be conducted in a consistent and reproducible manner.
Pediatric phantoms
In the past, we could “right-size” exams by dialing down the mAs based on patient size. So, if we delivered 180 mAs for an average-sized patient, we could simply adjust mAs based on patient size and weight, provided everything else about the protocol stayed the same. However, this is not typically the case. Pitch values, detector collimations, and image reconstruction kernels are varied, depending on the diagnostic task and patient size (for example, thinner slices are typically required in small children).
Thus, instead of simply scaling by mAs, exams should be “right sized” using a single standardized measure of scanner output, which takes into account the effects of tube potential, detector collimation, and pitch, etc. This is accomplished using CTDIvol, provided that the phantom sizes remain standardized. This is because the phantom size strongly affects the CTDI values. For example, using the 32 cm body CTDI phantom, our practice “dials down” by a factor of 5 between the typical adult CTDIvol values and what we use for a newborn. If however, CTDIvol values for children were measured using a smaller phantom (which on face value seems to make sense), the factor by which we dial down changes. For the exact same scanner setting, but using a large phantom with adult CTDIvol measurements and a small phantom for children, the “dial down factor” changes from 5 to 2. Thus, in order to provide helpful guidance about “right sizing” scanner output, the phantom size must be unambiguous.
Unfortunately, there is ambiguity in the current situation. The international safety standards relevant to CT specify that the 32 cm body phantom be used to specify scanner output, regardless of the size of patient. However, not all manufacturers adhere to this. Additionally, the pediatric DRL from the American College of Radiology (ACR) CT Accreditation Program is based on the 16 cm phantom. The professional and industry organizations involved in CT dosimetry standardization efforts are aware of this ambiguity and are working to adopt a fully consistent system in the near future.
Discussing dose with patients
While extremely useful for managing the dose applied to the patient, CTDIvol and effective dose values are not particularly helpful when talking to patients and counseling them with regard to benefit vs. risk decisions. Rather, CTDIvol and effective dose are tools that help us to know if our systems are operating correctly and if our doses are consistent with those of our colleagues.
When discussing risks with patients, it is important that the information be presented positively and in a context that is most helpful in making an informed decision. The considerable amount of alarmist media attention to radiation dose issues is, unfortunately, leading to the situation where patients are declining important diagnostic exams that could have immediate benefit for managementof their medical care simply based on what they have heard about “CT scans causing cancer.”
Because of the generally limited education provided in our public schools regarding radiation, many common misconceptions occur. For example, the irradiation of a pregnant patient that is necessary for management of the mother’s medical care presents a considerably smaller risk to the developing child than is commonly presumed. When counseling a pregnant woman about the risk of radiation, she should first understand the baseline risk of, for example, a malformation; in the absence of any radiation exposure, there is a 96% likelihood of giving birth to a healthy child. This likelihood changes negligibly, to 95.98%, after exposure of the fetus when the mother receives a typical abdomen and pelvis CT. Compared to the risk of an unnecessary appendectomy or missing an organ laceration subsequent to maternal trauma, the risk of the CT is negligible compared to the potential health benefits.
Because there is so much uncertainty in risk estimates at the low doses associated with medical exposures, it makes sense to stratify risk into relatively broad categories. For a chest radiograph, the risk of cancer is approximately 1 in 1,000,000. For a multiphase CT exam, the risk increases by a factor of 1,000, but is still extremely low (approximately 1 in 2,000). Thus, even though the CT exam maybe the equivalent of 500 chest X-rays, the estimated risk of the CT exam is still extremely low, particularly compared to the 1-in-4 chance each person has, in the absence of any radiation exposure, of dying from cancer. These risk estimates are conservative; below 100 mSv, it has not been scientifically demonstrated that there is a statistically significant increase in risk. However, for the purpose of erring on the side of safety, radiological professionals choose to assume that the risk does exist. Accordingly, we have the mandate to minimize risk by keeping doses as low as reasonably achievable (consistent with accomplishing the diagnostic task).
Conclusions
Comparison of risk between imaging exams should be performed between exams offering similar information content. For example, a cardiac CT exam should be compared to an invasive coronary angiogram or a nuclear medicine cardiac stress test; these exams are all relevant to cardiac health. The information content of a thoracic CT exam is orders of magnitude greater than that of a chest X-ray and thus, assuming the medical indication for the exam requires more information than a chest X-ray can provide, the CT exam is far more likely to benefit the patient in the near term compared with potentially harming them over the long term (a decade or more).
Modern CT technology continues to reduce the amount of radiation required to deliver high-resolution images for an ever-increasing amount of noninvasive imaging applications. It is incumbent upon professionals in the imaging community to optimize exam protocols and to continue to invest in user education and dose-reduction technology. However, if a CT is ordered for a medically appropriate reason, we are obligated to reassure the patient that the potential benefit to their health far outweighs the very small risk associated with ionizing radiation. Imaging professionals should “right size” the radiation output according to the patient’s size and shape, and the diagnostic task, and CT practices should benchmark their typical protocols against those of their colleagues using standardized dosemetrics such as CTDIvol. We should encourage and facilitate our technologists’ education as CT technology evolves, offering more advanced and complicated features.
These steps ensure that patients receive the lowest dose appropriate to an exam indication. While fulfilling this obligation, we must continue to be wary and speak out against inappropriate use of CT. But, amongst all these activities, we must not forget the inestimable value that CT offers to patients, expediting accurate and noninvasive diagnoses and guiding therapeutic decisions and procedures. The vast amount of media coverage regarding radiation dose has certainly facilitated greater awareness and education within the imaging community. However, these alarmist reports are scaring some patients away from needed medical examinations, and that simply is not good medicine.
REFERENCES
- Shope TB, Gagne RM, Johnson GC. A method for describing the doses delivered by transmission X-ray computed tomography. Med Phys. 1981;8:488-495.
- Jessen KA, Shrimpton PC, Geleijns J, et al. Dosimetry for optimisation of patient protection in computed tomography. Appl Radiat Isot. 1999;50: 165-172.
- European Commission EUR 16262 (2000) European Guidelines on Quality Criteria for Computed Tomography. Available at: http://drs.dk/guidelines/ct/quality. Accessed on Sept.24, 2009.
- Shrimpton PC. Appendix C. In: Bongartz G, Golding SJ, Jurik AG, et al. Eds. European guidelines for multislice CT. Funded by the European Commission. Contract No. FIGM-CT2000-20078-CT-TIP. 2004.
- Shrimpton PC, Hillier MC, Lewis MA, Dunn M. National survey of doses from CT in the UK: 2003. Br J Radiol. 2006;79:968-980. Erratum in: Br J Radiol. 2007;80:685. Dosage error in article text. Shrimpton, et al., Br J Radiol. 2006;79:968-980.
- CJ Martin. Effective dose: How should it be applied to medical exposures? Br J Radiol. 2007; 80:639-647.