CT and radiation: What radiologists should know
Images
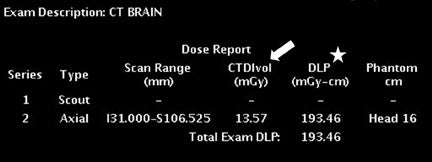
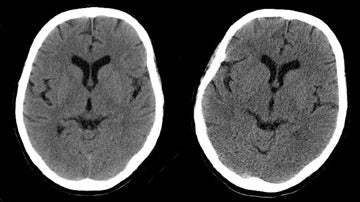
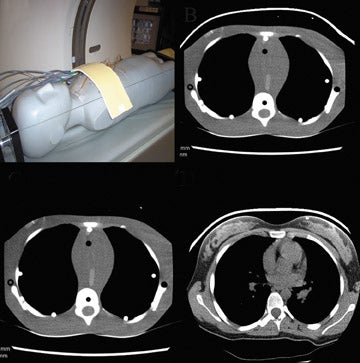
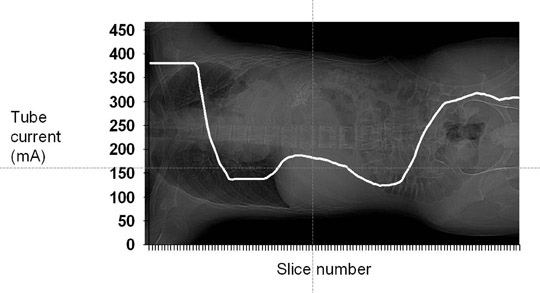
Dr. Coursey is a Radiology Resident, and Dr. Frush is a Professor of Radiology and Pediatrics and the Director of the Division of Pediatric Radiology, Department of Radiology, Duke University Medical Center, Durham, NC.
It is estimated that >60 million computed tomography (CT) examinations were performed in the United States in 2005.1 With a current U.S. population of just >300 million, this equates to 1 CT per year for 20% of the U.S. population, or, over the course of 5 years (with stable population numbers) 1 CT for every U.S. citizen. As faster CT scanners with increasing numbers of detector arrays are developed and dual energy/dual source technologies are increasingly available (along with the new CT protocols that are necessary), we are constantly challenged to find methods for CT dose reduction (such as tube current modulation). In addition, the use of CT may outpace science, which shows that the technology actually has a cost-effective benefit. In short, there is increasing pressure to depend on CT for diagnosis and a lack of guidance for how to best perform this examination.
Therefore, the fundamental goal of this article is to help radiologists make thoughtful decisions about radiation dose—ie, the quantity of radiation delivered to a patient with a given CT examination—just as a primary care physician would think about the dose of antibiotic prescribed or as a radiologist would think about the dose of intravenous contrast delivered.2 This understanding of dose then can serve as a guide when deciding about ranges of acceptable image quality.
To this end, this article reviews scanner-based CT radiation dose estimations and why CT radiation dose is generally of more concern than dose delivered by other diagnostic imaging modalities, presents a summary of the cancer risk of radiation doses delivered by CT, outlines parameters contributing to CT radiation dose, and describes techniques for reducing CT radiation dose. While the material applies to young adults, these objectives will be illustrated primarily through pediatric CT. Furthermore, most of the discussion will focus on illustrations of multidetector CT (MDCT), although many principles will apply to all clinical CT technology.
Scanner-based CT radiation dose estimation
Two related measures of CT radiation dose are available on CT consoles: the CT dose index (CTDI) and the dose length product (DLP)3,4 (Figure 1). The CTDI represents the radiation dose of a single CT slice and is determined using acrylic phantoms.3 These acrylic phantoms are cylinders of a standard length and are generally in diameters of 16 cm and 32 cm.
As defined by the Food and Drug Administration (FDA) in 1984, the original incarnation of the CTDI was based on an axial CT scanner.4 This original definition of CTDI represented the dose from the primary beam plus scatter from surrounding slices. Several variations of the CTDI have since been defined. For example, the CTDI100 reflects the dose contribution from a 100-mm range centered on the index slice. The weighted CTDI (CTDIw) reflects the weighted sum of two thirds peripheral dose and one third central dose in a 100-mm range in acrylic phantoms. The volume CTDI (CTDIvol), defined as CTDI divided by the beam pitch factor, is the most commonly cited index for modern MDCT equipment.4
The dose length product (DLP) is the CTDIvol multiplied by the scan length (slice thickness × number of slices) in centimeters. It should be noted that the DLP is independent of what is actually scanned. In other words, the reported DLP is the same whether a 10-lb infant or a 100-lb teenager is scanned if the scan length and other scan parameters are the same. Conversion factors can be used to estimate what the effective dose equivalent would be. However, these conversion factors are problematic in that they are only estimates of dose and do not represent the full range of pediatric sizes.
In order to determine a more accurate effective dose equivalent, individual organ doses would have to be determined, which would be impossible during clinical MDCT. The effective dose equivalent is the sum of the product of organ doses (in mGy or cGy, the magnitude of CT organ doses) multiplied by a corresponding weighting factor.5 The effective dose equivalent, therefore, represents a total body dose. For regional exposures, the effective dose equivalent is the equivalent dose to the whole body, for example, approximately
2.0 to 3.0 mSv for a head CT. In conclusion, the DLP method for estimating dose is problematic and offers only an approximation. However, this method is of value from the standpoint of ease of use and as a gauge for dose in one’s practice.
Why is CT radiation dose potentially so high?
There are several reasons why CT radiation dose is potentially high: 1) there is no dose penalty for relatively high radiation dose examinations; 2) CT doses are intrinsically high radiation dose examinations; 3) there are “hidden” dose penalties that occur with CT; and (4) there is no binding regulation for CT practice.
There is no compromise in image quality for relatively high-dose CT examinations. Compare this to the setting of film screen radiography (and even, to some extent, digital radiography), in which an overexposed film (and therefore an “overdosed” patient) is relatively straightforward to identify—the film is too black. However, with CT technology, patients can receive extremely high doses of radiation without a dramatic change in image quality that would signal to the radiologist that the patient has been overexposed or “overdosed.” However, in most cases, this information is available to the radiologist in the form of image annotations (eg, tube current) and information provided on the CT console (eg, the DLP) (Figure 1).
CT radiation doses can be quite high. While doses, especially in pediatric CT, can be <1.0 mSv, doses can be >30 mSv as well (unpublished data, CL Hollingsworth, MD, Durham, NC; Radiological Society of North America 2004). The effective dose of a chest CT (eg, 5 mSv) is nearly 100 times the effective dose from a frontal and lateral chest radiographic series (0.06 mSv) in an adult.6 When settings are not adjusted for size, CT doses are higher in small children. For example, the effective dose of a chest CT in an infant can be 2 to 3 times the effective dose of a chest CT in an adult if the settings are not adjusted for size.7 The potential doses delivered by newer CT technologies can be quite high. For example, using a 5-year-old anthropomorphic phantom on a 64-slice CT scanner and maximizing settings to deliver the highest dose possible, we were able to perform an abdomen and pelvis CT examination that resulted in a dose of slightly less than 120 mSv (unpublished data; Donald P. Frush, MD, Duke University Medical Center, Durham, NC). This dose is beyond the range of low-level radiation dose and is at or approaching medium-level exposure, at which there is a clear connection with cancer risk.
In the United States, the data regarding the overall contribution of CT to radiation exposure are compelling. Traditionally, it has been thought that approximately 80% of all radiation exposure comes from background sources and 15% is from medical radiation, with up to 67% due to CT (or roughly 10% of the total radiation dose). However, the contribution from CT to the total radiation dose to the U.S. population has probably been underestimated. Given a background radiation dose of approximately 3.0 mSv per year, if 10% is due to CT, it should contribute roughly 0.375 mSv per person per year. However, we can also approach this from the standpoint of CT examinations performed currently. Assuming that there are 60 million CT examinations performed annually in the United States with a population of approximately 300 million people, 1 CT is performed for every 5 individuals. If we assume that a single CT, irrespective of the region scanned, delivers 6.0 mSv (head and chest CT may be <5.0 mSv, whereas abdomen scanning is often >8.0 mSv), then 1 in 5 individuals (20% of the population) will receive twice the annual background dose of 3.0 mSv in a single CT examination. Spreading this out, 100% of the population, on average, will receive an additional 40% of background dose per year, or an additional 1.2 mSv. Contrast this to the previous estimate of 0.375 mSv, and the amount actually received from CT alone is larger by a factor of 3.2; and the relative contributions to exposure are 66% as a result of background and 26% as a result of CT alone. At the April 2006 National Council on Radiation Protection and Measurement Annual Meeting in Washington, D.C., preliminary data suggested that the contribution of CT to total radiation dose exposure could be approximately 50%.8
“Hidden doses” of radiation also add to the dose delivered by CT. For example, each time an additional phase is performed, it results in an additional dose. In addition, as the effective beam becomes larger (eg, currently 40 mm for some scanners), there is some dose that is not accounted for in image formation since some gantry rotation must be completed before there are sufficient data to begin image formation. For a small range scanned (eg, a child), this can result in a higher dose than displayed.9 Moreover, the CTDI and DLP do not account for newer scan technologies with larger effective beam widths.10,11 Basically, the scatter radiation now extends well beyond the measurement range and is therefore not accounted for in calculations of the CTDI and DLP. In support of this, we found an underestimation using the DLP method of effective dose by as much as 35% in certain body MDCT protocols in the adult female.12
Despite the potentially high radiation doses CT can deliver, there is no regulation of CT practice in the United States. Regulation is up to the individual practitioner. While the American College of Radiology has a CT accreditation program, which includes upper limits of doses for CT, participation in this accreditation is voluntary at this point in time. This is in contrast to other countries and regions. In the United Kingdom, medical exposure ionizing radiation regulations were initiated in 2000.13 As part of this regulation, it is the responsibility of radiologists to perform only those examinations that are thought to be justified, and radiologists are granted the authority to refuse any studies that are not appropriate.14
Cancer:The bioeffect of concern with CT
Two points about cancer risk and low-level radiation dose, such as that from CT, are worth mentioning. First, whether or not radiation doses at levels delivered by CT produce cancer remains a controversial topic. Second, radiation dose is a greater concern in children.
Regarding the first point, there is strong support for a linear, nonthreshold model of radiation dose in which any radiation dose is thought to increase one’s risk of developing cancer.15 On the other hand, others argue that low doses of radiation (including the levels delivered by CT) are harmless or may actually be therapeutic (eg, stimulate the immune system). This is the concept of hormesis through stimulation of the immune system.16
While most of the emphasis on the potential radiation dose dangers is in the pediatric population, the issue applies to adults as well. In one example in support of cancer risk and CT, Brenner et al17 looked at screening CT in adults using a linear model based on atomic bomb survivor data in the specific scenario of a 45-year-old man who undergoes a screening chest, abdomen, and pelvis CT every year for 30 years and computed an estimated lifetime attributable cancer mortality risk of approximately 0.08% for a single examination and of about 1.9% for 30 examinations; radiation-induced lung cancer was the dominant cause of cancer mortality.17
Breast radiation dose is also worth mentioning, given the association between breast radiation dose and breast cancer. It has been estimated that a dose of 0.01 Gy (1.0 cGy) to the breast of a woman <35 years of age increases her risk of breast cancer by approximately 14% over the expected spontaneous rate for the general population.18 A recent investigation by Hurwitz et al19 reported breast doses of 4 to 6 cGy for a standard pulmonary embolism protocol CT (140 kVp, 380 mA, 0.8-sec rotation, 16 × 1.25 collimation), 1 to 2 cGy for a standard appendicitis protocol CT (140 kVp, 340 mA, 0.5-sec rotation, 16 × 0.625 collimation), and 150 μGy for a standard renal calculus protocol CT (140 kVp, 160 mA, 0.5-sec rotation, 16 × 0.625 collimation) using metal oxide semiconductor field effect transistor (MOSFET) detector technology and a female-configured anthropomorphic phantom.19 As the use of chest CT is increasing (including evaluation for pulmonary embolism and cardiac evaluation or screening) and the use of CT increases in younger populations, these radiation doses will need to be carefully considered as a factor in the complex risk-benefit balance.
The second point is that CT dose and potential risk is of special importance in pediatric patients because of their much larger increase in lifetime risk per unit radiation dose, greater sensitivity of organs and tissues, and relatively greater dose deposition compared with adults from similar CT settings.20-23 For example, Brenner et al24 estimated a lifetime cancer mortality risk attributable to a single CT (with relatively high dose) of 0.18% for an abdomen pelvis CT and 0.07% for a head CT protocol performed in a 1-year-old child.
Parameters contributing to CT radiation dose
The principal selectable parameters that contribute to radiation dose are tube current (mA), peak kilovoltage (kVp), pitch, and gantry cycle time (in seconds) (Table 1). The relationship between tube current and radiation dose is linear. Decreasing tube current by 50% will essentially decrease radiation dose by 50%.25 In contrast to the relationship between tube current and dose, the relationship between kilovoltage and dose is nonlinear.26 For example, when all other parameters were held constant with a single-slice CT scanner, when kVp was increased from 120 to 140 (a 17% increase), the CTDIw increased by 37.5% for a head phantom and 39% for a body phantom.26
Pitch (defined as table distance traveled in one 360° rotation/total collimated width of the X-ray beam) is inversely proportional to patient dose. Larger pitches lower the radiation dose. The relationship between pitch and radiation dose is linear. Specifically, increasing the pitch from 1.0 to 1.5 will reduce the patient dose by 33%.5 Most body CT scanning, especially in children, is performed at pitches between approximately 1.0 to 1.5.
Decreasing gantry rotation time decreases radiation dose in a linear fashion.27 The faster the gantry rotation, the lower the dose. Increasing the cycle speed of rotation from 1.0 to 0.5 seconds per 360° rotation reduces the dose essentially by 50%. Of course, when these variables are adjusted to decrease dose, the tradeoff is an increase in image noise. The increase in noise that resulted when tube current was decreased from 280 to 140 mA is illustrated in Figure 2.
Techniques for decreasing radiation dose
Most efforts at reducing dose through selectable parameters are focused on tube current (including using tube current modulation) and kVp. Additional strategies include minimizing multiphase scanning, limiting the range of coverage, and using in-plane shielding. As always, optional imaging modalities that do not expose the individual to radiation or provide additional substantive risks, such as magnetic resonance imaging or sonography, should be considered.
Extensive work has been done, primarily in the pediatric population, to develop CT protocols that are based on the patient’s size. For example, pediatric guidelines have been published that discuss size-based, lower-dose scanning for specific applications.2,28-31 There are also investigations that support the use of relatively lower tube currents for pediatric CT of the brain,32-34 sinuses,35,36 tracheobronchial tree,37 chest,38-40 pelvis,41 skeletal system,42 and colon (colonography).43 Rogalla et al39 concluded that age-adjusted tube currents from 25 to 75 mA (using a 1-second gantry rotation time) were of diagnostic quality.
In a study of CT examinations submitted for review at a tertiary care center in the southeastern United States, Paterson et al44 reported that many referring physicians were not adjusting scan parameters for pediatric patients. Prompted by increased awareness of the detrimental effects of radiation on pediatric patients and information that CT parameters were not being adjusted for pediatric patients, in 2002 the FDA issued a Public Health Notification entitled “Reducing radiation risk from computed tomography for pediatric and small adult patients,” which encouraged optimizing CT settings (reducing tube current, increasing pitch, and developing a chart of tube current settings based on patient size and anatomical region of interest), reducing the number of multiple scans with contrast material (for example, eliminating unnecessary precontrast scans), and eliminating inappropriate referrals for CT when other modalities, such as ultrasound or MRI, could be performed instead of CT.45
Recent data suggests that practice patterns are, in fact, changing. In April 2007, data was presented at the Society for Pediatric Radiology annual meeting that represented a 5-year–interval survey of pediatric body MDCT use by the membership. Approximately 40% of respondents indicated using a kVp of <110 in 2006 versus <5% in 2001 (P <0.0001). In addition, the mean mA for CT in the 0-to-4-year age group decreased from >120 mA to approximately 70 mA in abdomen CT and from approximately 110 mA to 50 mA in chest CT. Both of these changes in MDCT mA practice are also statistically significant.
Whenever possible, multiphase scanning should be eliminated. When settings are not adjusted, each study will result in a dose that is a multiple of the number of phases performed. In our practice, multiphase scanning is not part of our routine protocols, should be performed on a case-by-case basis, and should account for up to only roughly 5% of pediatric body CT protocols.
Other techniques for dose reduction include bismuth shielding and automatic tube current modulation (ATCM). Bismuth shielding has been shown to reduce radiation dose while still producing diagnostic quality images (Figure 3). Bismuth breast shields have been shown to reduce breast dose by 26.9% to 52.4% in the adult population depending on the thickness of the shield.46 Similarly, bismuth breast shields have been shown to reduce breast dose by 29% in pediatric patients.47 Bismuth shielding has also been shown to reduce direct radiation dose to the orbits by 34%.48 At our institution, bismuth breast shields are generally used when scanning women <50 years of age when breast tissue is included in the range of scanning and in select cases in pediatric scanning. Our experience is that 2-ply shields can be used in girls who have not yet undergone breast development, after which 4-ply shielding is more appropriate. Pediatric breast shields are now available.49
Automatic tube current modulation also can be used to decrease radiation dose. The 3 primary means of ATCM include angular (x- and y-axis), longitudinal (z-axis), and combined (x-, y-, and z-axis) modulation. With angular modulation, relatively higher tube currents are delivered through the thicker region of an ellipse (e.g, mediolateral abdomen) as compared with the thinner dimension (e.g, anteroposterior abdomen). With z-axis modulation, tube current is altered along the craniocaudal dimension of the patient delivering lower tube currents through less attenuating structures (e.g, the lungs) and relatively higher tube currents through more attenuating structures (e.g, the shoulders). The technical basis that determines the modulation varies by manufacturer and was recently summarized by McCollough et al.50 Figure 4 illustrates the use of one manufacturer’s tube current modulation technique (Auto mA and GE LightSpeed 16-slice CT, GE Healthcare, Waukesha, WI); the modulation along the z-axis is based on the density of tissues seen on the topogram (scout image). As seen in Figure 4, relatively higher tube currents are delivered through the shoulders (peak 381 mA) and pelvis (peak 318 mA). Relatively lower tube currents are delivered through the lungs (nadir 137 mA) and lower abdomen (nadir 125 mA). For this type of modulation, the technologist selects a noise target. Tube currents are then modulated (within a selected maximum and minimum range) to maintain the selected noise index.
Dose savings with ATCM can be quite substantial. In the setting of pediatric chest CT, Greess et al51 found dose reductions of 26% to 43% when ATCM was used (dose decrease depended on the patient’s geometry and weight) as compared with standard weight-adapted protocols. In the setting of adult chest CT, z-axis ATCM has been shown to decrease radiation dose by 18% to 26% when the selected noise indices were 10.0 and 12.5 HU, respectively.52 For adult abdomen pelvis CT, z-axis ATCM has been shown to reduce mean tube current-time product by 31.9% (range 18.8% to 87.5%) as compared with fixed tube current scanning.53 Combined ATCM (x-, y-, and z-axis modulation) in the setting of adult abdomen pelvis CT has been shown to decrease dose by 43%.54
In our work investigating ATCM with Auto mA and the LightSpeed 16-slice CT scanner and 2-ply bismuth pediatric breast shields (F & L Medical Products, Vandergrift, PA) in the setting of pediatric chest MDCT, the highest dose saving (52%) was achieved when the shield was placed after the scout image was performed.49 When the shield was present in the scout radiograph, ATCM compensated for the presence of the shield by increasing tube current through the level of the shield, minimizing dose savings through that region. Despite this increase, doses in the scans using ATCM and breast shields were still lower than in scans performed with the standard, age-based protocol.
How are these variables reconciled into practical CT protocols? There are a few simple guidelines that can be followed. Thin adults can be scanned at lower tube currents than heavier adults. This principle applies to pediatric scanning, where a variety of sources provide recommendations for size-based scanning parameters.2,29-31 Higher contrast regions or organs lend themselves to both lower tube current and lower kVp.55,56 These regions include lung parenchyma, skeletal system, and some CT angiography. Tube current modulation, when properly applied, is useful for dose reduction and should be used when doses will not be higher than when a nonmodulated examination is performed. This necessitates familiarity with the specific technology used in one’s practice. In short, to lower the radiation dose (assuming the CT examination is indicated and the scanning range is properly defined), one should always consider lowering the tube current and the kVp, scanning with a relatively large pitch, and using shielding when appropriate. Finally, educational and regulatory efforts are important venues for balancing radiation dose and image quality. Many of these efforts and additional recommendations were recently outlined in the ACR White Paper on Radiation Dose in Medicine.57
Image quality considerations
The goal of dose management should not always be dose reduction. A balance must be struck between dose and image quality. The image quality portion of this balance is much more intangible in nature than the dose side. Basically, image quality has physical, or objective, properties—such as mottle, contrast, or artifacts—and subjective properties. The objective properties related to the parameters discussed above are measurable. Subjective qualities also define the diagnostic yield of a CT scan. These subjective factors often take into account the structure or region being assessed. For example, higher mottle may be tolerated in relatively high contrast areas such as lung parenchyma or the skeletal system. Desired image quality also depends on the nature of the disorder being addressed. Assessment for trauma might lend itself to higher mottle than evaluation of small hepatic metastases. In an investigation of adults with acute abdominal pain reported at the 2007 ARRS Meeting, Udayasankar et al58 reported that very (“ultra”) low-dose CT (120 kVp, 25 to 100 mAs with tube current modulation) resulted in both a sensitivity and negative predictive value of 93%. Ultra-low-dose CT was shown to have a sensitivity of 92.8% with a negative predictive value of 92.6% in adults in the setting of abdominal pain. Image quality also depends on individual preferences, which may be based on experience (ie, training) in addition to institutional, community, national, and medical specialty societal or organizational guidelines.59
Conclusion
It has been estimated that 1 CT scan was performed for every 5 Americans in 2005, and this number is expected to continue to increase. While the issue of whether or not radiation doses at levels delivered by CT can cause cancer is debated, the ALARA (As Low As Reasonably Achievable) principle is not. Extensive work in the pediatric radiology community has shown that we do not need to and, in fact, should not be performing CT examinations of infants with the same scan parameters as CT examinations of teenagers. Substantially lower tube current settings, and lower kVp, for example, can be used in smaller patients and still yield diagnostic quality images with dose savings. Likewise, in adult radiology, we do not need to image a small adult with the same scan parameters we use for a large or obese adult to obtain diagnostic quality images. However, work in the small, medium, and large categories in adult CT has yet to be as pervasive as the size-based scanning in children.
In addition to size/weight-based protocols, automatic tube current modulation and bismuth breast shields have each been shown to decrease radiation dose. Because there are no federal regulations as of yet in the United States regarding CT imaging and radiation dose, it is up to individual radiologists and radiology departments to control the radiation dose that patients receive. As radiologists, we have the responsibility to properly “dose” our patients. Ignoring this responsibility is, in the authors’ estimation, medical error.
REFERENCES
- Levatter RE, Brenner DJ, Elliston CD. Radiation risk of body CT: What to tell our patients and other questions [Letter to the Editor]. Radiology. 2005;234:968-970.
- Frush DP. Strategies of dose reduction. Pediatr Radiol. 2002;32:293-297.
- Lai KC, Frush DP. Managing the radiation dose from pediatric CT. Appl Radiol. 2006;35(April suppl);13-20.
- Yoshizumi TT, Nelson RC. Radiation issues with multidetector row helical. Crit Rev Comput Tomogr. 2003;44:95-117.
- Huda W, Slone R. Review of Radiologic Physics. 2nd ed. Baltimore, MD: Lippincott, Williams & Wilkins; 2003.
- Wall BF, Hart D. Revised radiation doses for typical X-ray examinations. Report on a recent review of doses to patients from medical X-ray examinations in the UK by NRPB. National Radiological Protection Board. Br J Radiol. 1997;70:437-439.
- Huda W. Effective doses to adult and pediatric patients. Pediatr Radiol. 2002;32:272-279.
- U.S. exposure to medical radiation soars, ACR issues new white paper on dosage. Diag Imag Intell Rep. June 2007. Available online at: http://www.g2reports.com/issues/DIIR/2007_6/1612608-1.html.
- Thomton FJ, Paulson EK, Yoshizumi TT, et al. Single versus multi-detector row CT: Comparison of radiation doses and dose profiles. Acad Radiol. 2003;10: 379-385.
- Dixon RL. A new look at CT dose measurement: Beyond CTDI. Med Phys. 2003;30:1272-1280.
- Brenner DJ. : Is it time to retire the CTDI for CT quality assurance and dose optimization [Letter to the Editor]? Med Phys. 2005;32:3225-3226.
- Hurwitz LM, Yoshizumi TT, Goodman PC, et al. Effective dose determination using an anthropomorphic phantom and metal oxide semiconductor field effect transistor technology for clinical adult body multidetector array computed tomography protocols. J Comput Assist Tomogr. 2007;31:544-549.
- Department of Health Web Site (United Kingdom). The Ionising Radiation (Medical Exposure) Regulations 2000 (together with notes on good practice). Available at: http://www.health-carecommission.org.uk /serviceproviderinformation/irmer2000.cfm. Accessed: June 8, 2007.
- Walker A, Tuck JS. Ionising Radiation (Medical Exposure) Regulations: Impact on clinical radiology. Br J Radiol. 2001;74:571-574.
- Pierce DA, Preston DL. Radiation-related cancer risks at low doses among atomic bomb survivors. Radiat Res. 2000;154:178-186.
- Cohen BL. Cancer risk from low-level radiation. AJR Am J Roentgenol. 2002;179:1137-1143.
- Brenner DJ, Elliston CD. Estimated radiation risks potentially associated with full-body CT screening. Radiology. 2004;232:735-738.
- Remy-Jardin M, Remy J. Spiral CT angiography of the pulmonary circulation. Radiology. 1999;212:615-636.
- Hurwitz LM, Yoshizumi TT, Reiman RE, et al. Radiation dose to the female breast from 16-MDCT body protocols. AJR Am J Roentgenol. 2006;186: 1718-1722.
- Khursheed A, Hillier MC, Shrimpton PC, Wall BF. Influence of patient age on normalized effective doses calculated for CT examinations. Br J Radiol. 2002; 75:819-830.
- Huda W, Scalzetti EM, Levin G. Technique factors and image quality as functions of patient weight at abdominal CT. Radiology. 2000;217:430-435.
- Hall EJ. Lessons we have learned from our children: Cancer risks from diagnostic radiology. Pediatr Radiol. 2002;32:700-706.
- Pierce DA, Shimizu Y, Preston DL, et al. Studies of the mortality of atomic bomb survivors. Report 12, Part I. Cancer:1950-1990. Radiat Res. 1996;146:1-27.
- Brenner DJ, Elliston CD, Hall EJ, Berdon WE. Estimated risks of radiation-induced fatal cancer from pediatric CT. AJR Am J Roentgenol. 2001;176:289-296.
- Frush DP, Donnelly LF, Rosen NS. Computed tomography and radiation risks: What pediatric health care providers should know. Pediatrics. 2003;112:951-957.
- McNitt-Gray MF. AAPM/RSNA Physics Tutorial for Residents: Topics in CT: Radiation dose in CT. RadioGraphics. 2002;22:1541-1553.
- Smergel E, Benson D. Radiation dose on pediatric CT: Losing track of time [Letter]. AJR Am J Roentgenol. 2002;178:507-508.
- Donnelly LF, Frush DP. Pediatric multidetector body CT. Radiol Clin North Am. 2003;41:637-655.
- Donnelly LF, Emery KH, Brody AS, et al. Minimizing radiation dose for pediatric body applications of single-detector helical CT: Strategies at a large Children’s Hospital. AJR Am J Roentgenol. 2001;176: 303-306.
- Frush DP. Pediatric body CT. In: Knollman F, Coakley FV, eds. Multislice CT: Principles and Protocols. Philadelphia, PA: Elsevier, Inc.; 2005: 179-201.
- Frush DP. Pediatric CT: Practical approach to diminish the radiation dose. Pediatr Radiol. 2002; 32:714-717; discussion 751-754.
- Cohnen M, Fischer H, Hamacher J, et al. CT of the head by use of reduced current and kilovoltage: Relationship between image quality and dose reduction. AJNR Am J Neuroradiol. 2000;21:1654-1660.
- Huda W, Lieberman KA, Chang J, Roskopf ML. Patient size and x-ray technique factors in head computed tomography examinations. II. Image quality. Med Phys. 2004;31:595-601.
- Huda W, Lieberman KA, Chang J, Roskopf ML. Patient size and x-ray technique factors in head computed tomography examinations. I. Radiation doses. Med Phys. 2004;31:588-594.
- Hein E, Rogalla P, Klingebiel R, Hamm B. Low-dose CT of the paranasal sinuses with eye lens protection: Effect on image quality and radiation dose. Eur Radiol. 2002;12:1693-1696.
- Mulkens TH, Broers C, Fieuws S, et al. Comparison of effective doses for low-dose MDCT and radiographic examination of sinuses in children. AJR Am J Roentgenol. 2005;184:1611-1618.
- Pacharn P, Poe SA, Donnelly LF. Low-tube-current multidetector CT for children with suspected extrinsic airway compression. AJR Am J Roentgenol. 2002;179:1523-1527.
- Lucaya J, Piqueras J, García-Peña P, et al. Low-dose high-resolution CT of the chest in children and young adults: Dose, cooperation, artifact incidence, and image quality. AJR Am J Roentgenol. 2000; 175:985-992.
- Rogalla P, Stöver B, Scheer I, et al. Low-dose spiral CT: Applicability to paediatric chest imaging. Pediatr Radiol. 1999;29:565-569.
- Ambrosino MM, Genieser NB, Roche KJ, et al. Feasibility of high-resolution, low-dose chest CT in evaluating the pediatric chest. Pediatr Radiol. 1994;24:6-10.
- Kamel IR, Hernandez RJ, Martin JE, et al. Radiation dose reduction in CT of the pediatric pelvis. Radiology. 1994;190:683-687.
- Salamipour H, Jimenez RM, Brec SL, et al. Multidetector row CT in pediatric musculoskeletal imaging. Pediatr Radiol. 2005;35:555-564.
- Anupindi S, Perumpillichira J, Jaramillo D. Low-dose CT colonography in children: Initial experience, technical feasibility, and utility. Pediatr Radiol. 2005;35:518-524.
- Paterson A, Frush DP, Donnelly LF. Helical CT of the body: Are settings adjusted for pediatric patients? AJR Am J Roentgenol. 2001;176:297-301.
- Food and Drug Administration. FDA public health notification: Reducing radiation risk from computed tomography for pediatric and small adult patients. Pediatr Radiol. 2002;32:314-316.
- Hopper KD, King SH, Lobell MH, et al. The breast: In plane x-ray protection during diagnostic CT—shielding with bismuth radioprotective garments. Radiology. 1997;205:853-858.
- Fricke BL, Donnelly LF, Frush DP, et al. In-plane bismuth breast shields for pediatric CT: Effects on radiation dose and image quality using experimental and clinical data. AJR Am J Roentgenol. 2003;180: 407-411.
- Perisinakis K, Raissaki M, Theocharopoulos N, et al. Reduction of eye lens radiation dose by orbital bismuth shielding in pediatric patients undergoing CT of the head: A Monte Carlo study. Med Phys. 2005;32:1024-1030.
- Coursey C, Frush DP, Yoshizumi T, et al. Pediatric chest MDCT and tube current modulation: Effect on radiation dose with breast shielding. AJR Am J Roentgenol. 2008;190:W54-W61.
- McCollough CH, Bruesewitz MR, Kofler JM, Jr. CT dose reduction and dose management tools: Overview of available options. RadioGraphics. 2006;26:503-512.
- Greess H, Lutze J, Nömayr A, et al. Dose reduction in subsecond multislice spiral CT examination of children by online tube current modulation. Eur Radiol. 2004;14:995-999.
- Kalra MK, Rizzo S, Maher MM, et al. Chest CT performed with z-axis modulation: Scanning protocol and radiation dose. Radiology. 2005;237:303-308.
- Kalra MK, Maher MM, Toth TL, et al. Comparison of z-axis automatic tube current modulation technique with fixed tube current CT scanning of the abdomen and pelvis. Radiology. 2004;232:347-353.
- Rizzo S, Kalra M, Schmidt B, et al. Comparison of angular and combined automatic tube current modulation techniques with constant tube current CT of the abdomen and pelvis. AJR Am J Roentgenol. 2006;186:673-679.
- Sigal-Cinqualbre AB, Hennequin R, Abada HT, et al. Low-kilovoltage multi-detector row chest CT in adults: Feasibility and effect on image quality and iodine dose. Radiology. 2004;231:169-174.
- Schueller-Weidekamm C, Schaefer-Prokop CM, Weber M. CT angiography of pulmonary arteries to detect pulmonary embolism: Improvement of vascular enhancement with low kilo-voltage settings. Radiology. 2006;241:899-907.
- Amis ES, Jr., Butler PF, Applegate KE, et al. American College of Radiology white paper on radiation dose in medicine. J Am Coll Radiol. 2007;4:272-284.
- Udayasankar UK, Li J, Kalra MK, et al. Ultra-low-dose MDCT of abdomen and pelvis in patients with acute nonspecific abdominal pain: Impact on patient care and management. Abstract AJR Am J Roentgenol. 2007;188:A27-A29
- Frush DP. Radiation dose and image quality for pediatric CT: Clinical considerations. Categorical Course in Physics presented at the Radiological Society of North America, Chicago, IL, November 28, 2006.