Digital Radiography: Evolving Technologies, Definitions, and Applications
Images
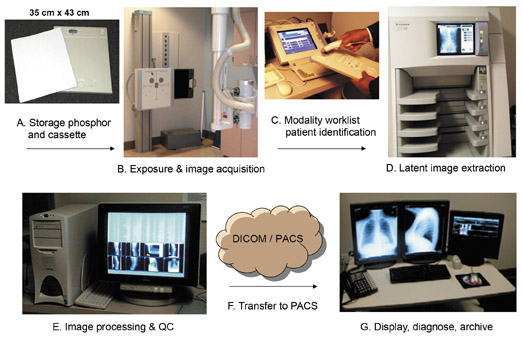
Is there a sense of unjustified prejudice in digital X-ray imaging? This segment of radiographic imaging has experienced double-digit market growth during the past decade, and there is an expanding, diverse range of technology available. But are these emerging devices inappropriately classified based on preconceived notions? Is it time to reexamine our perceptions and to consider a logical means of understanding, describing, and using future digital projection radiography technologies?
As a modality, digital radiography (DR) has been segregated into systems labeled DR , computed radiography (CR), digital , direct , and indirect , as well as other names. Unfortunately, these labels often increase confusion. Within these broad labels, there is a great diversity of technology, with specific strengths and weaknesses. For instance, a variety of detectors are used within the category of DR and, within CR, different imaging plates and scanning methods are used. Using these labels, assumptions have been made as to imaging performance, diagnostic capabilities, and workflow efficiencies. Instead, each system should be evaluated on its own merits. Perhaps it is time to take a step back and begin an open-minded discussion of the realities of this technology.
This supplement to Applied Radiology contributes to such a discussion. These informative articles present a range of material on the evolution of DR, distinctions in the teminology and technology, how to select systems to meet an institution's needs, and what the future may hold for digital imaging.
In supporting this publication, it is our hope that readers will explore the evolution of digital X-ray terms and categories, which will help them to better understand the differences and similarities between the technologies. It is important for radiologists and radiology administrators to consider, evaluate, and categorize digital X-ray systems based on how they actually address and satisfy their departments' needs.
John B. Strauss
Director of Marketing, Imaging Systems
FUJIFILM Medical Systems USA, Inc.
Stamford, CT
Dr. Seibert is a Professor of Radiology, University of California, Davis Medical Center, Sacramento, CA.
Categorizing digital radiography systems is no longer as simple as "computed radiography" (CR) and "direct and/or digital radiography" (DR). The more technology changes, the more it changes. The CR versus DR issue is no exception, where historical boundaries of CR (with passive, cassette-based image acquisition and detector handling with offline processing) and the fully integrated DR system (with automatic processing and display) are now converging. Historically, CR has referred to the implementation of a cassette-based photostimulable storage phosphor (PSP) imaging plate reader and quality-control workstation that are packaged as an add-on system to existing X-ray devices using screen-film detectors. In contrast, DR has been touted as a totally integrated X-ray source-generator-detector solution with images displayed for review within the room shortly after the X-ray exposure. Now, "CR" technology (specifically using PSP converters) has been developed into integrated X-ray systems and has completely automated acquisition, display, and processing. Now "DR" technology has cassette-based detectors available for situations requiring more flexibility in positioning and multiple uses in conventional X-ray cassette trays and bedside portable radiography.
Computed radiography uses a PSP that transiently stores a latent image in the form of electrons in semistable traps within the phosphor structure, with subsequent data extraction using a stimulating point laser beam that scans the phosphor surface point by point. Line scan laser sources coupled to an array of microlens and photodiode light detectors stimulate and acquire data in parallel, reducing the readout time of a PSP imaging plate from about a minute to ≤10 seconds, which is comparable to other digital radiography detectors known for readout speed.
In its current definition, DR describes a multitude of digital X-ray detection systems that immediately process the absorbed X-ray signal after exposure and produce the image for viewing with no further user interaction. This fact has resulted in the use of the term direct radiography by many manufacturers, which has added to the nomenclature confusion, because of another use of direct that describes the conversion of X-rays into charge, which is described later. In terms of CR versus DR, inclusion of automated CR systems would fall into the DR category, along with optically coupled scintillator to charge-coupled device (CCD) camera systems, fiberoptically coupled rectangular CCD array slot-scan detector systems, complementary metal-oxide semiconductor (CMOS) detector systems, thin-film-transistor (TFT) flat-panel detector systems, and slot-scan photon counting detectors, which were recently introduced. The CR and DR acronyms no longer define the essence of digital radiography detector attributes, since distinct classification into these 2 broad categories is no longer possible and, in fact, often leads to confusion and "marketeering" claims and counterclaims.
In a recent publication by a multi-societal effort (including the American College of Radiology, the American Association of Physicists in Medicine, and the Society of Imaging Informatics in Medicine) to determine practice guidelines for the use of CR and DR in the clinical environment, 1 a consensus to title the work Practice Guidelines for Digital Radiography 2 was made to be inclusive of all types of digital radiographic systems, in recognition of the issues explained above. Likewise, in this article, the term digital radiography refers to all types of digital radiographic systems, including both those that had been historically termed CR and those historically termed DR .
So, how should one categorize current state-of-the-art digital radiography technology? One method promoted in this article considers 3 characteristics: 1) detector form factor; 2) image acquisition geometry; and 3) X-ray signal conversion method, as explained below. Various digital radiography detectors and attributes are listed in Table 1.
Detector form factor considers aspects such as "cassette" versus "cassetteless" (Figure 1) and "passive" versus "active" operation. A majority of cassette-based digital detectors are based on PSP technology as a direct replacement for screen-film, thus providing a cost-effective means to achieve digital image acquisition, while at the same time allowing great positioning flexibility. Passive operation allows an asynchronous coupling of the X-ray exposure and storage of the image signal on the phosphor. Subsequent processing is performed by physically inserting the cassette/ phosphor plate into an image "reader" to render the radiograph. Multiplexing of several rooms to 1 reader is possible but is also time-ineffi-cient and potentially limiting in busy, high-throughput rooms. An alternative is a cassette-based TFT-array detector for use with portable radiography examinations and as a "drop-in" to cassette trays in general radiographic rooms. This detector uses a wired electronic connection to the X-ray generator to actively integrate and read out the X-ray image after exposure. A CMOS detector in a cassette configuration for mammography is available as a replacement for screen-film mammography. Wireless interfaces will likely soon appear on such detector systems. Image display shortly after the exposure and good positioning flexibility are benefits; damage to the detector by unintentional mishandling is a potentially costly drawback.
Cassetteless digital detectors are part of an integrated X-ray generator, X-ray tube, and detector system. The basic meaning of "cassetteless" indicates that the image is acquired and subsequently displayed at the in-room technologist console for review and manipulation with minimal user interaction. The earliest "cassetteless" systems had PSP plate changers with automated acquisition, point-scan laser readout, image processing, and display, with individual processing times on the order of a minute. Recent introduction of line-scan laser excitation of the PSP imaging plate reduces readout time of a large 35 × 43-cm field-of-view (FOV) detector in as few as 5 seconds, with automatic processing and display. This is comparable to many large FOV optically coupled CCD and flat-panel TFT arrays, which comprise the majority of cassetteless digital radiography systems. In the extreme are "real-time" flat-panel systems specifically manufactured for fluoroscopic imaging that also provide radiographic capabilities. Other cassetteless radiographic systems include slot-scan acquisition devices in which the image is produced by scanning a collimated beam and detector array across the FOV over a time span of seconds.
Image acquisition geometry distinguishes instantaneous acquisition of a large-area digital image with a short exposure time versus a sequential, long exposure time acquisition of a slot-scan device. Most digital detectors for radiography use a large-area FOV geometry, which allows short exposure times to decrease the probability of patient motion. However, detected scatter from the patient reduces image contrast and consumes a fraction of the digital range of the detector with essentially useless information. Thus, an antiscatter grid is often used with thicker body parts (it is used in essentially all adult imaging except extremity examinations) and in-curs a relatively large dose penalty (typically double the patient dose) because of the loss of primary radiation. The slot-scan geometry with pre-and post-patient collimation produces a narrow fan-beam incident on a scintillator coupled to a time-delay-integrate CCD photodetector array. 3 Since at any instant only a small fraction of the patient volume is irradiated, the amount of scatter is reduced to low levels, and the amount of detected scatter is extremely low because of postpatient collimation and a small detector area. Grids are not required, which results in good dose utilization; image acquisition times, however, are extended into seconds, and the detector/collimator configuration makes positioning a challenge for nonstandard imaging protocols.
X-ray signal conversion is described by indirect, direct, or photon-counting methods. All digital detectors produce an output signal, usually in the form of electrons or holes (positive ions), which represent a quantity of charge that is proportional to the number of Xrays ab-sorbed at a specific detector element (del) position. The magnitude of the charge is converted to a voltage and then to a digital value for storage in the image matrix at the corresponding del location in the detector plane.
Indirect refers to the conversion of X-rays into secondary information carriers, such as absorbed X-ray energy to light energy conversion in a scintillator, or to stored electrons in semistable traps within a storage phosphor and subsequent stimulation with a laser and light emission. For each X-ray photon absorbed, a large amplification of light photon carriers is produced on account of the large energy difference of X-rays (keV) versus light (eV) or energy required to trap electrons in a storage phosphor (eV). Regardless of the method by which secondary light photons are formed, the large numbers are directed to a wavelength-matched sensitive photodiode-TFT array via direct optical coupling, to a CCD area detector by lens-optical coupling, or via a light-guide pickup to a photomultiplier tube in a PSP reader device. Charge amplitude is generated in response to the amplitude of light intensity (and thus X-ray intensity). A proportional voltage is produced and digitized to form the digital gray-scale image. Thus, scintillator-based TFT arrays, optically coupled CCD camera systems, and fiberoptically coupled CCD slot-scan systems as well as all PSP systems are classified as indirect acquisition devices.
Direct , in the context of this article (unlike the use of this term by many manufacturers that produce indirect acquisition DR devices), refers to the method of ac-quisition and conversion of absorbed X-ray energy into electron/hole pairs (charge) using semiconductor converters such as amorphous selenium ( a -Se), solid-state silicon, or high-pressure gas. These direct acquisition detectors have a voltage applied to electrodes on opposite surfaces of the absorber/semiconductor material to separate and collect the generated electrons and holes. A small amount of energy (on the order of 50 eV) is needed to produce an ion pair for a -Se; for a 50-keV absorbed X-ray, hundreds of charge information carriers are generated. Similar amplification of charge per absorbed X-ray photon occurs in other direct converters as well. Electric field lines direct the ion pairs to the collection electrodes without lateral spreading, thus providing high intrinsic resolution by accurately mapping the X-ray photon absorption event to the corresponding del position.
Photon-counting detectors are currently configured in slot-scan geometry, composed of either high-pressure gas or solid-state silicon. These detectors measure absorbed X-ray photon events individually as counts instead of energy integration like all other detectors. 4 Since a count will be tallied independent of the photon energy, a signal-to-noise ratio advantage of up to 40% is achieved for the same number of X-ray photons absorbed in the detector compared with energy integration detectors, as there is no bias toward higher energy photons, and elimination of other noise sources accompanying an energy-integrator detector is possible. A limitation is the maximum count rate that can be sustained.
So, what is the "best" digital radiography detector? There is no straightforward answer, as advantages and disadvantages are based upon multiple comparisons of the following characteristics: detective quantum efficiency (DQE), spatial resolution, contrast resolution, dose efficiency, acquisition and display speed, radiographic positioning flexibility, image quality, ability to use existing radiographic equipment, integration with information systems and electronic networks, system costs, service costs, detector longevity, and survival in a hostile environment (eg, trauma imaging), among many other benchmarks. Matching an appropriate digital radiography device with its intended clinical purpose is an exercise in determining actual needs and patient throughput requirements prior to evaluating the type of DR system that will best meet those needs in a cost-effective and efficient manner. 5,6
Conclusion
The terms CR and DR will continue to be used, but collectively we must think beyond the traditional CR versus DR comparisons, past the issues of X-ray converter materials, to clinical relevance, cost-effectiveness, dose efficiency, image processing functionality, overall image quality, proper use of digital radiography attributes (eg, variable speed characteristics and dose index values), quality-control phantoms and automated computer routines to verify proper function, patient throughput, uptime, reliability, longevity, service, and
optimization in the clinical arena. Insisting on DR systems that can self-monitor and verify optimal performance through the automated analysis of quality-control phantom images will move the industry toward providing these important tools.
Finally, while dose efficiency is important, it shouldn't be the overriding consideration in determining the type of imaging system that best meets a specific clinical need, as all DR systems that are approved by the U.S. Food and Drug Administration must demonstrate a reasonable image quality over a typical range of incident exposures for clinical procedures. More important is an understanding and use of exposure index values that describe the "effective speed" of the detector to ensure that overexposures that are otherwise difficult to discern do not become the standard of practice as a result of complacency and/or ignorance.
REFERENCES
- Williams MB, Krupinski EA, Strauss KJ, et al. Digital radiography image quality: Image acquisition. J Am Coll Radiol.2007;4:371-388.
- ACR Practice Guideline for Digital Radiography. Approved May 2007. Available on the American College of Radiology Web site at: http://www.acr.org/ SecondaryMainMenuCategories/quality_safety/guidelines/MedicalPhysics.aspx. Accessed August 8, 2007.
- Samei E, Lo JY, Yoshizumi TT, et al. Comparative scatter and dose performance of slot-scan and full-field digital chest radiography systems. Radiology. 2005; 235:940-949.
- Pisano ED, Yaffe MJ. Digital mammography.Radiology.2005;234:353-362.
- Reiner BI, Siegel EL, Hooper FJ, et al. Multi-institutional analysis of computed and direct radiography: Part I. Technologist productivity. Radiology.2005;236: 413-419.
- Reiner BI, Salkever D, Siegel EL, et al. Multi-institutional analysis of computed and direct radiography: Part II. Economic analysis. Radiology.2005;236:420-426.
Mr. Romlein is a Managing Partner of Qualiteering Labs LLP, Thurmont, MD.
In 1990, projection radiography comprised roughly 70% of the total imaging workload of radiology departments; currently it accounts for just over 50%. Computed radiography (CR) was the first large-scale technology to support digital projection radiography, but it has now been complemented by digital radiography (DR). One key underlying reason for the different names was originally based upon the use of either cassette-based photostimulable phosphor imaging plates (CR) or integrated solid state devices (DR) as the X-ray image receptors. While there were clear distinctions in early CR and DR, the two technologies have grown toward each other in their efforts to satisfy the needs of the projection radiography workflow, throughput, and feature requirements.
Over time, there has been constant market pressure in the development of the two modalities to make improvements in what was seen to be the shortcomings of both CR and DR. These gradual design changes caused the differences between the 2 technologies to begin to disappear as they each moved toward becoming a better and more versatile modality in support of the projection radiography marketplace.
Blurred lines of distinction
A key early distinction between CR and DR was the use of centralized versus decentralized workflow. As originally implemented, CR was a cassette-based, centralized system that mimicked the workflow model of a conventional film-based radiology department using a daylight loading film processor. The centralized design was a good fit for the existing layout of the radiology work cores, which allowed for an easy transition of the film processing spaces and, even more importantly, the cassette-based CR design required no modification of the X-ray machines themselves.
The concept of a decentralized workflow, in which image acquisition, image inspection and adjustment, and transfer to the picture archiving and communication system (PACS) could be accomplished by the technologist without ever leaving the X-ray room, was not even a consideration in the early days. Such a workflow model was never available for general radiology applications in the film-based era; it would have required a daylight loader in each room. The closest approximation to a decentralized film-based system was the dedicated chest room with an automatic film changer.
On the other hand, DR, in the form in which it was originally implemented, required, and still requires a decentralized workflow. The DR detector was permanently associated with the X-ray generator and tube head. Each DR room operated as an independent system. Each system was associated with a single-operator console from which the images are acquired, assembled into a completed study, adjusted as necessary, and transferred to either the PACS or a laser film printer.
Distinctions and blur factors
The following paragraphs list a series of commonly believed distinctions between CR and DR technologies and offer a description of how that distinction has been blurred by changes in the technologies or their applications.
Distinction: Cassette-based systems require that the technologist transport cassettes to a centralized processing location because the physical facility cannot accommodate a decentralized workflow.
Blur factor: CR cassette readers have decreased in size and cost and have increased in speed, so that it is now feasible to have a dedicated reader inside each X-ray room or control booth, which enables a decentralized workflow that is very similar to a DR system.
Distinction: CR systems all use cassette-based photostimulable phosphor (PSP) detector image capture with associated human transport to a centralized CR reader, image processing, and DICOM transfer station.
Blur factor: Current cassetteless PSP-based X-ray systems incorporate the image receptor into the design of the X-ray systems with no human transport of the image receptor required.
Distinction: CR is required to obtain digital radiographs from portable X-ray systems.
Blur factor: Portable X-ray units are now available with an integrated "tethered" DR detector that requires no human transport to a CR reader and processing station following the exposure.
Distinction: The integrated DR detector on a portable X-ray unit has the advantage over CR of giving the technologist feedback on positioning and image quality while still at bedside.
Blur factor: Portable X-ray units are now available with integrated CR cassette readers that approach this same functionality and require minimal human transport.
Distinction: Certain projections are difficult to obtain on DR systems due to the bulk of the DR detector/bucky and the physical limitations of the detector suspension systems.
Blur factor 1: DR rooms can now be configured as hybrid systems with both suspended bucky detectors and tethered flat-panel detectors. This provides the additional freedom to use the tethered detector as a free cassette for difficult positioning situations.
Blur factor 2: A cassetteless PSP integrated X-ray system could exhibit the same positioning limitations as a DR-integrated X-ray system.
Distinction: Research has shown significant differences in total study performance time principally because of the human transport of the CR cassettes and postprocessing workflow. 1
Blur factor: While this is true for most cassette-based, centrally organized CR systems, the PSP-integrated X-ray systems eliminate all human transport requirements, use high-speed line-scanning technology for image acquisition, and consolidate the inspection, processing, and DICOM transmission functions into the X-ray control area, thus reducing or eliminating the workflow time differences.
Detectors
Several different digital detectors have emerged and have been put into production for different digital imaging uses. For the most part, there is little the consumer can do to influence which detector type is used in any given device, but some basic understanding of the differences is helpful.
CR generally implies the use of PSP detectors, which are typically used in cassette-based imaging applications and are now found in cassetteless digital imaging systems. Commercial detectors have been introduced based on PSP compounds including but not limited to: rubidium chloride (RbCl), and a variety of barium flourohalides doped with europium (BaFBr:Eu 2 , BaF(Br,I):Eu 2 , and BaFI:Eu). These are unstructured phosphors that are scanned in a raster pattern using laser light of a specific wavelength. The resulting light emissions escaping from the phosphor are concurrently collected and measured by either a moving photo detector or a light collection circuit that feeds a stationary photo detector. The collected light signals are proportional to X-rays captured on the imaging plate and so have the relative spatial and contrast information required to reconstruct the X-ray image. This scanning methodology and BaFBr imaging plates are used in cassette-based readers and have been incorporated into some cassetteless X-ray systems. These cassetteless systems also incorporate a rapid imaging plate (IP) changing mechanism to rotate between multiple installed IPs. This technique makes a blank IP rapidly available for the next exposure while scanning the IP that was last exposed to X-rays.
Cesium bromide doped with europium (CsBr:Eu) is a newer phosphor that is sometimes referred to as a needle phosphor because of its needlelike structure. This PSP is more efficient in its return of signal per unit of X-ray dose and can be scanned by a line-scanning mechanism, which reduces the image acquisition cycle to <10 seconds. CsBr receptors work well in cassetteless X-ray systems because of the very high image transfer rate.
DR generally implies the use of solid-state detectors that were initially integrated into a cassetteless relationship with an X-ray source, generator, and control station. The use of solid-state imaging systems in cassette-based image receptors has recently been introduced but is limited to a subset of detector types and imaging systems. DR systems use arrays or flat panels that are made up of thin-film transistor (TFT) arrays or of other detector materials. The structure of the array must accommodate either the direct or indirect X-ray conversion properties of each detector material to produce electrical output signals proportional to the received X-ray image.
General radiography and digital mammography systems use the widest variety of digital detectors including, but not limited to, the following types: amorphous silicon TFT (a-Si TFT), amorphous selenium TFT (a-Se TFT), charge-coupled device (CCD), and complementary metal oxide semiconductors (CMOS). All but one (a-Se TFT) of these detector types use indirect conversion processes to change received X-rays into electrical signal. Digital detectors that are typically used for acquiring fluoroscopic imagery include a-Si TFT and a-Se TFT. Digital detectors made of Gd 2 O 2 S photodiode TFT arrays and CMOS detectors are exceptions, as they have been used in cassette-based systems.
In general, these arrays are made up of discrete detector elements (dels) arranged into a flat panel. Each del, either directly or indirectly, builds up an electrical charge that is proportional to the amount of X-rays striking it. Each del is also directly addressable via the imbedded circuitry of the flat panel and can be read following the X-ray exposure. Reading out the electrical signals sequentially in lines and rows reproduces the spatial arrangement of the flat panel and delivers an image matrix of discrete picture elements (pixels), each of which is proportional to a small portion of the X-ray image.
Spatial resolution
Spatial resolution is dependent on several factors. In DR receptors, the actual size of the dels in the image receptor matrix coupled with the geometry of the X-ray system and the patient establishes a spatial relationship to the anatomy being imaged. The closer the centers of the dels are, the higher the spatial resolution. In arrays that use indirect DR detectors, the amount of light spread during the X-ray-to-light conversion process has a negative effect on spatial resolution. To limit this, some manufacturers use structured converters that contain the emitted light and reflect it toward a single del. PSP receptors do not have physical image elements and instead rely on photo detectors being rapidly sampled during the motion of the IP through the detector mechanism. The faster the sample rate, the higher the resulting spatial resolution, as long as the signal-to-noise ratio remains acceptable.
Motion-related artifacts
A key difference between PSP detectors and most digital detectors is that PSP image acquisition and erasure is accomplished by some form of relative motion between the image phosphor and the light collection and erasure circuitry. This relative motion can be the source of artifacts for a variety of reasons. 2 Most solid-state detectors (with the exception of the slot-scanned detectors used in some applications) do not use any moving parts to acquire the images from the receptors or to perform erasures and are, therefore, free of this source of artifact.
DR receptor artifacts
The solid-state image receptors have a set of issues that PSP receptors do not. All dels must be periodically equalized to provide the same base state for an image to be built upon. Additionally, some dels will fail to respond for one reason or another and must be masked from their surrounding dels to prevent dead pixels from appearing in the displayed image. While masking methods vary, the general process is to create a map of all failed dels and upon image processing, paint the image pixels that correspond to the failed dels with approximations of the signals from their surrounding pixels, thus masking the failing dels.
Exposure indicators
Film-screen quality control operations monitored X-ray exposure as it was indexed to the optical density (OD) of processed films. While most CR manufacturers provide an indicator of how much X-ray exposure arrived at the imaging receptor, there is no consistency in the methods used or in the scale of the indicators. For the most part, DR manufacturers do not express exposure index at all. The American Association of Physicists in Medicine (AAPM) Task group 116 has begun work on this issue and has recommended a uniform exposure indicator for digital imaging. This recommendation has not yet been implemented in the industry.
Consistency versus best of breed
In the analog film/screen days, we usually worked with a standard film/ screen combination throughout the de-partment, in order to have a consistent appearance. There is an advantage to keeping each diagnostic modality area on similar imaging platforms; this preserves the consistency of workflow functionality and the look and feel of images. Such consistency reduces mistakes and retakes and optimizes the comparison of images over time. The best-of-breed approach for selecting CR and DR imaging systems often mixes systems of different types and adds confusion and inconsistency to an imaging section's operations and the resulting imagery.
Conclusion
In the ongoing discussion of DR versus CR, while there are differences in the underlying technologies, there is a growing overlap in their functionality and performance levels. The use of the term "digital radiography" to mean both DR and CR makes sense, given that digital imaging operations are so similar and must be addressed collectively as well as individually.
Adding the terms "cassetteless" and "cassette-based" to the conversation (as is suggested by the American College of Radiology, 3 AAPM, and the Society for Imaging Informatics in Medicine) adds even more clarity. Understanding and retaining the details of the actual acquisition devices and their image receptors is, of course, important for a variety of reasons. These include performing valid acceptance testing and quality control and retaining a valid history and audit trail in our libraries, technical documentation, and imaging archives. Additionally, while the lines between DR and CR have been blurred, the study of underlying technologies associated with each exposes their differences, such as motion artifacts and lack of exposure indices, which should be understood and managed in order to optimize imaging operations. Finally, the overmixing of imaging devices with different technologies into a single workflow center can lead to confusion, errors, waste, and inconsistent imagery.
REFERENCES
- Reiner BI, Siegel EL, Hooper FJ, et al. Multi-institutional analysis of computed and direct radiography: Part 1. Technologist productivity. Radiology. 2005;236:413-419.
- Willis CE, Thompson SK, Shepard SJ. Artifacts and misadventures in digital radiography.Appl Radiol.2004:33(1):11-20.
- ACR. Practice Guideline for Digital Radiography. (Res. 42) October 1, 2007. Reston, VA: American College of Radiology. 2007:23-35. Available in pdf format at www.acr.org/SecondaryMainMenuCategories/quality_safety/guidelines/dx/digital_radiography.aspx. Accessed October 3, 2007.
Ms. Dallessio is the Technology Correspondent for Applied Radiology.
As radiology workloads continue to increase and the staffing shortage shows no signs of abating, the choice of X-ray and other imaging equipment becomes an important factor in determining how effectively and productively a radiology department can function. When purchasing new equipment, the stakeholders in the imaging department must carefully consider many factors, including workflow, image quality and consistency, ease of use, connectivity, quality assurance, service, maintenance, and acquisition and usage costs.
"In any equipment purchase, you need to look at the clinical utility of what you are trying to achieve. Then you must brace that against the quality of the product and the quality of the outcome you are trying to achieve: in this case, images and imaging," said Steven Metcalf, BS, CRA, Manager of Radiology Services, Altru Health System, Grand Forks, ND.
"In our department, when it came time to purchase new X-ray equipment, we weighed the image quality versus the utility of the equipment versus the overall cost of the equipment. Then, given our budgetary restraints, we decided what would make the most sense," said Russell E. McWey, MD, Chief of Medical Imaging, Virginia Hospital Center, Arlington, VA. "Then we made on-site visits and looked at the individual pieces of equipment to help us decide what we liked and did not like."
Workflow efficiencies
For many radiology departments, workflow efficiency is the primary consideration when purchasing new X-ray equipment. "Generally, workload trends are always on the increase in radiology. I do not think that is unique to my service. It is a well-identified national trend," said Metcalf. "In addition, I believe the radiologist shortage will continue to plague us for some time to come. Certainly, the implementation of digital technology and picture archiving and communications systems (PACS) is a benefit because of the way they improve efficiency."
The issue of workflow was also an important consideration for Radiology Manager Mark Brown, BA, RT(R)(M) and his coworkers when they were asked to purchase X-ray equipment for Memorial Health Systems' new Memorial Hospital North in Colorado Springs, CO. "The first consideration was how we could decrease the number of full-time equivalents (FTEs) and still offer state-of-the-art imaging," said Brown, "because I knew we were going to have a limited number of FTEs available for the new hospital."
"In today's digital world," said Metcalf, "there are efficiencies that a particular product may bring to your department, and with digital radiology (DR) products that is certainly a factor. Workflow is a major concern, particularly as it relates to the implementation of DR, specifically what this technology can do based on the fact that the user is not handling cassettes and the technologist does not need to be involved in processing images."
"With DR," explained Brown, "the technologist does not have to touch the cassette or process anything. The machine does it automatically. The images are immediately transferred to a monitor, where the user can manipulate them and prepare them for the radiologist. DR gives you all the information at one time. The technologist can position the patient on the table, take the exposure, and, by the time he or she is ready to position the patient for the next exposure, the first exposure is ready to be reviewed and manipulated."
Connectivity
How well the new equipment interacts with a facility's information infrastructure is critical to the purchasing decision. "Connectivity was a huge issue for us as well," said Brown. "With our last system, we had several connectivity issues and concerns. The biggest factor in this is the PACS because it is system-wide and everyone is affected by it. When we first implemented PACS in 2000, connectivity was a nightmare. We ended up buying black boxes to place everywhere when we had been under the assumption that the system was just 'plug and play.'"
"The implementation of PACS was a driver in our purchasing decision," added Metcalf. "In the PACS world, you want to move all equipment into the digital modality. With radiographic services, that means the implementation of computed radiography (CR) or DR. In our facilities, we used a combination of CR and DR and placed DR systems into each of our 3 main facilities where we have the highest volume. In the other locations, it made more sense for us to put in CR. In our main hospital, we have a DR suite, as well as single-plate reader systems in our intensive care unit (ICU) and other high-usage areas among the nursing floors."
The issue of connectivity was also very important at Virginia Hospital Center, according to McWey. He noted that they considered how the data would be processed and stored and which processing algorithms can be applied to the image at the reading station when it is retrieved on the PACS.
Learning curve and the user interface
Along with connectivity, when migrating to digital systems, the consistency of the user interface and the learning curve should be considered. It takes time for technologists to become proficient with a new system. "When you move the technologist from the traditional world of analog imaging to digital, it's not as easy a transition as people think it might be," explained Brown. "It is important to be sure that the technologists are prepared for the change," he advised. "If the new system takes them out of their comfort zone, then they will be a bit more apprehensive. There is a large learning curve that can't be ignored." When the user interface is consistent and familiar, the transition can be easier and, said Metcalf, "your staff training is more uniform, and you have fewer errors with those functions."
"When we switched to DR at our institution, there was certainly a learning curve in understanding the exposure differences between analog imaging and DR and CR," said Metcalf, "but the technologists transitioned quite smoothly."
"I think it was easier since the interfaces were similar to what the technologists had been using before," added McWey. "So even though it was a new product and had to be learned, our new DR system has the same look as the old CR systems in terms of how patient information is input and the protocols are selected."
For Brown, the opening of a new campus was an opportunity to bring in new technologists and train them immediately on the new technology. "All of the diagnostic radiology equipment at our outpatient facilities is DR-based," he explained. "So I thought this was a great opportunity for us to go totally DR at the new campus and to move the newly hired technologists to DR from the start. That way we wouldn't have to retrain them on DR once we upgraded."
Image quality and consistency
The main function of any radiology department is to provide clear, easy-to-read images that help the physician make an accurate diagnosis. Therefore, the quality and consistency of the images produced should be of prime importance when choosing new radiology equipment. "Image resolution is extremely important," said McWey.
One of the advantages of DR in terms of image quality, he noted, is the fact that DR is a closed system; therefore, the risk of artifacts due to contamination with dirt or dust is reduced. "Since DR is a contained system," he said, "there are very few issues with artifact contamination."
"There is not a lot of Quality Assurance (QA) work required for this system," continued McWey. "It's all integrated in the system. The only maintenance we perform is to erase the receptors daily. Routine maintenance is performed by the manufacturer."
"In the applications training process for the technologists, quality control was addressed," added Metcalf. "The technologists were taught how to ensure--by looking at the data screens when they capture an image--that they have the correct exposure and that the image is of proper quality."
At Memorial Hospital North, they decided to institute a new QA program when they installed their new DR system. "Every morning we image a phantom to make sure that when the radiologists pull images up, they are consistent," explained Brown. "We use the same technique, the same density, and the same resolution to be able to check the images."
Cost and service concerns
"There are other factors that also come into play when choosing new equipment," said Metcalf, "including the support of your regional sales and service force and costs. Certainly a lower cost was important to us, although it wasn't a huge issue."
"For us, service was a huge concern," said Brown. "If the manufacturer doesn't have adequate service, it doesn't do us any good. We can't function effectively if the machine goes down."
In addition to the acquisition cost, the ongoing cost of consumables is also a consideration in choosing the right equipment for your facility. With DR, there are fewer consumable costs than with analog equipment. "You don't have to purchase film or the chemistry products and equipment that are needed to process the film," said Metcalf. "In addition, there are no cassettes to be replaced either."
Making the right decision
When it comes to making the final purchase decision, all of the stakeholders in the process should be involved. "At our facility, the radiologists were very involved when it came to the PACS decision, primarily from the aspect of selecting the workstations and understanding the functionality of the workstations, because they are their tools," said Metcalf. "The DR decision, on the other hand, was pretty much left to the technical staff. For vendor selection, we primarily used our technical staff and technical supervisors," explained Metcalf. "I was more involved in the financial analysis and assessing quotes and bids to ensure that they were competitive in the market."
"We have a committee that made the final decision regarding which PACS system we purchased," said Brown. "After that, the radiology management team sat down and decided which equipment would fit best in the areas we manage. Most of the time, it's a team effort through which we poll the staff to see what they need. Then the managers and directors have their input. No single individual makes the final decision."
In Virginia, the decision was made by McWey and the radiology administrator. "We had some input from the general radiologists, but it was primarily the two of us, our PACS administrator, and our radiology information system (RIS) administrator who were involved in the decision-making process."
"We narrowed the choice down to 2 or 3 vendors," concluded Metcalf. "The FUJI SpeedSuite (FUJIFILM Medical Systems USA, Stamford, CT) was a very attractive product for us in that it bridged the gap when it came to comparing CR to DR in the workflow. It also bridged the gap for us in relation to the cost comparison with the more traditional type of DR system with the amorphous flat-panel silicone detector. In fact, when we began our selection process," he said, "we did not think we were going to choose DR. We expected to implement CR throughout the facility. However, this particular product gave us the opportunity to think about our DR decision again. The decisive factor was that we could improve our workflow process with DR in our 3 high-volume areas. Once we realized that, it became a very good and obvious choice for us."
Dr. Seibert is a Professor of Radiology, University of California, Davis Medical Center, Sacramento, CA.
Categorizing digital radiography systems is no longer as simple as "computed radiography" (CR) and "direct and/or digital radiography" (DR). The more technology changes, the more it changes. The CR versus DR issue is no exception, where historical boundaries of CR (with passive, cassette-based image acquisition and detector handling with offline processing) and the fully integrated DR system (with automatic processing and display) are now converging. Historically, CR has referred to the implementation of a cassette-based photostimulable storage phosphor (PSP) imaging plate reader and quality-control workstation that are packaged as an add-on system to existing X-ray devices using screen-film detectors. In contrast, DR has been touted as a totally integrated X-ray source-generator-detector solution with images displayed for review within the room shortly after the X-ray exposure. Now, "CR" technology (specifically using PSP converters) has been developed into integrated X-ray systems and has completely automated acquisition, display, and processing. Now "DR" technology has cassette-based detectors available for situations requiring more flexibility in positioning and multiple uses in conventional X-ray cassette trays and bedside portable radiography.
Computed radiography uses a PSP that transiently stores a latent image in the form of electrons in semistable traps within the phosphor structure, with subsequent data extraction using a stimulating point laser beam that scans the phosphor surface point by point. Line scan laser sources coupled to an array of microlens and photodiode light detectors stimulate and acquire data in parallel, reducing the readout time of a PSP imaging plate from about a minute to ≤10 seconds, which is comparable to other digital radiography detectors known for readout speed.
In its current definition, DR describes a multitude of digital X-ray detection systems that immediately process the absorbed X-ray signal after exposure and produce the image for viewing with no further user interaction. This fact has resulted in the use of the term direct radiography by many manufacturers, which has added to the nomenclature confusion, because of another use of direct that describes the conversion of X-rays into charge, which is described later. In terms of CR versus DR, inclusion of automated CR systems would fall into the DR category, along with optically coupled scintillator to charge-coupled device (CCD) camera systems, fiberoptically coupled rectangular CCD array slot-scan detector systems, complementary metal-oxide semiconductor (CMOS) detector systems, thin-film-transistor (TFT) flat-panel detector systems, and slot-scan photon counting detectors, which were recently introduced. The CR and DR acronyms no longer define the essence of digital radiography detector attributes, since distinct classification into these 2 broad categories is no longer possible and, in fact, often leads to confusion and "marketeering" claims and counterclaims.
In a recent publication by a multi-societal effort (including the American College of Radiology, the American Association of Physicists in Medicine, and the Society of Imaging Informatics in Medicine) to determine practice guidelines for the use of CR and DR in the clinical environment, 1 a consensus to title the work Practice Guidelines for Digital Radiography 2 was made to be inclusive of all types of digital radiographic systems, in recognition of the issues explained above. Likewise, in this article, the term digital radiography refers to all types of digital radiographic systems, including both those that had been historically termed CR and those historically termed DR .
So, how should one categorize current state-of-the-art digital radiography technology? One method promoted in this article considers 3 characteristics: 1) detector form factor; 2) image acquisition geometry; and 3) X-ray signal conversion method, as explained below. Various digital radiography detectors and attributes are listed in Table 1.
Detector form factor considers aspects such as "cassette" versus "cassetteless" (Figure 1) and "passive" versus "active" operation. A majority of cassette-based digital detectors are based on PSP technology as a direct replacement for screen-film, thus providing a cost-effective means to achieve digital image acquisition, while at the same time allowing great positioning flexibility. Passive operation allows an asynchronous coupling of the X-ray exposure and storage of the image signal on the phosphor. Subsequent processing is performed by physically inserting the cassette/ phosphor plate into an image "reader" to render the radiograph. Multiplexing of several rooms to 1 reader is possible but is also time-ineffi-cient and potentially limiting in busy, high-throughput rooms. An alternative is a cassette-based TFT-array detector for use with portable radiography examinations and as a "drop-in" to cassette trays in general radiographic rooms. This detector uses a wired electronic connection to the X-ray generator to actively integrate and read out the X-ray image after exposure. A CMOS detector in a cassette configuration for mammography is available as a replacement for screen-film mammography. Wireless interfaces will likely soon appear on such detector systems. Image display shortly after the exposure and good positioning flexibility are benefits; damage to the detector by unintentional mishandling is a potentially costly drawback.
Cassetteless digital detectors are part of an integrated X-ray generator, X-ray tube, and detector system. The basic meaning of "cassetteless" indicates that the image is acquired and subsequently displayed at the in-room technologist console for review and manipulation with minimal user interaction. The earliest "cassetteless" systems had PSP plate changers with automated acquisition, point-scan laser readout, image processing, and display, with individual processing times on the order of a minute. Recent introduction of line-scan laser excitation of the PSP imaging plate reduces readout time of a large 35 × 43-cm field-of-view (FOV) detector in as few as 5 seconds, with automatic processing and display. This is comparable to many large FOV optically coupled CCD and flat-panel TFT arrays, which comprise the majority of cassetteless digital radiography systems. In the extreme are "real-time" flat-panel systems specifically manufactured for fluoroscopic imaging that also provide radiographic capabilities. Other cassetteless radiographic systems include slot-scan acquisition devices in which the image is produced by scanning a collimated beam and detector array across the FOV over a time span of seconds.
Image acquisition geometry distinguishes instantaneous acquisition of a large-area digital image with a short exposure time versus a sequential, long exposure time acquisition of a slot-scan device. Most digital detectors for radiography use a large-area FOV geometry, which allows short exposure times to decrease the probability of patient motion. However, detected scatter from the patient reduces image contrast and consumes a fraction of the digital range of the detector with essentially useless information. Thus, an antiscatter grid is often used with thicker body parts (it is used in essentially all adult imaging except extremity examinations) and in-curs a relatively large dose penalty (typically double the patient dose) because of the loss of primary radiation. The slot-scan geometry with pre-and post-patient collimation produces a narrow fan-beam incident on a scintillator coupled to a time-delay-integrate CCD photodetector array. 3 Since at any instant only a small fraction of the patient volume is irradiated, the amount of scatter is reduced to low levels, and the amount of detected scatter is extremely low because of postpatient collimation and a small detector area. Grids are not required, which results in good dose utilization; image acquisition times, however, are extended into seconds, and the detector/collimator configuration makes positioning a challenge for nonstandard imaging protocols.
X-ray signal conversion is described by indirect, direct, or photon-counting methods. All digital detectors produce an output signal, usually in the form of electrons or holes (positive ions), which represent a quantity of charge that is proportional to the number of Xrays ab-sorbed at a specific detector element (del) position. The magnitude of the charge is converted to a voltage and then to a digital value for storage in the image matrix at the corresponding del location in the detector plane.
Indirect refers to the conversion of X-rays into secondary information carriers, such as absorbed X-ray energy to light energy conversion in a scintillator, or to stored electrons in semistable traps within a storage phosphor and subsequent stimulation with a laser and light emission. For each X-ray photon absorbed, a large amplification of light photon carriers is produced on account of the large energy difference of X-rays (keV) versus light (eV) or energy required to trap electrons in a storage phosphor (eV). Regardless of the method by which secondary light photons are formed, the large numbers are directed to a wavelength-matched sensitive photodiode-TFT array via direct optical coupling, to a CCD area detector by lens-optical coupling, or via a light-guide pickup to a photomultiplier tube in a PSP reader device. Charge amplitude is generated in response to the amplitude of light intensity (and thus X-ray intensity). A proportional voltage is produced and digitized to form the digital gray-scale image. Thus, scintillator-based TFT arrays, optically coupled CCD camera systems, and fiberoptically coupled CCD slot-scan systems as well as all PSP systems are classified as indirect acquisition devices.
Direct , in the context of this article (unlike the use of this term by many manufacturers that produce indirect acquisition DR devices), refers to the method of ac-quisition and conversion of absorbed X-ray energy into electron/hole pairs (charge) using semiconductor converters such as amorphous selenium ( a -Se), solid-state silicon, or high-pressure gas. These direct acquisition detectors have a voltage applied to electrodes on opposite surfaces of the absorber/semiconductor material to separate and collect the generated electrons and holes. A small amount of energy (on the order of 50 eV) is needed to produce an ion pair for a -Se; for a 50-keV absorbed X-ray, hundreds of charge information carriers are generated. Similar amplification of charge per absorbed X-ray photon occurs in other direct converters as well. Electric field lines direct the ion pairs to the collection electrodes without lateral spreading, thus providing high intrinsic resolution by accurately mapping the X-ray photon absorption event to the corresponding del position.
Photon-counting detectors are currently configured in slot-scan geometry, composed of either high-pressure gas or solid-state silicon. These detectors measure absorbed X-ray photon events individually as counts instead of energy integration like all other detectors. 4 Since a count will be tallied independent of the photon energy, a signal-to-noise ratio advantage of up to 40% is achieved for the same number of X-ray photons absorbed in the detector compared with energy integration detectors, as there is no bias toward higher energy photons, and elimination of other noise sources accompanying an energy-integrator detector is possible. A limitation is the maximum count rate that can be sustained.
So, what is the "best" digital radiography detector? There is no straightforward answer, as advantages and disadvantages are based upon multiple comparisons of the following characteristics: detective quantum efficiency (DQE), spatial resolution, contrast resolution, dose efficiency, acquisition and display speed, radiographic positioning flexibility, image quality, ability to use existing radiographic equipment, integration with information systems and electronic networks, system costs, service costs, detector longevity, and survival in a hostile environment (eg, trauma imaging), among many other benchmarks. Matching an appropriate digital radiography device with its intended clinical purpose is an exercise in determining actual needs and patient throughput requirements prior to evaluating the type of DR system that will best meet those needs in a cost-effective and efficient manner. 5,6
Conclusion
The terms CR and DR will continue to be used, but collectively we must think beyond the traditional CR versus DR comparisons, past the issues of X-ray converter materials, to clinical relevance, cost-effectiveness, dose efficiency, image processing functionality, overall image quality, proper use of digital radiography attributes (eg, variable speed characteristics and dose index values), quality-control phantoms and automated computer routines to verify proper function, patient throughput, uptime, reliability, longevity, service, and
optimization in the clinical arena. Insisting on DR systems that can self-monitor and verify optimal performance through the automated analysis of quality-control phantom images will move the industry toward providing these important tools.
Finally, while dose efficiency is important, it shouldn't be the overriding consideration in determining the type of imaging system that best meets a specific clinical need, as all DR systems that are approved by the U.S. Food and Drug Administration must demonstrate a reasonable image quality over a typical range of incident exposures for clinical procedures. More important is an understanding and use of exposure index values that describe the "effective speed" of the detector to ensure that overexposures that are otherwise difficult to discern do not become the standard of practice as a result of complacency and/or ignorance.
REFERENCES
- Williams MB, Krupinski EA, Strauss KJ, et al. Digital radiography image quality: Image acquisition. J Am Coll Radiol.2007;4:371-388.
- ACR Practice Guideline for Digital Radiography. Approved May 2007. Available on the American College of Radiology Web site at: http://www.acr.org/ SecondaryMainMenuCategories/quality_safety/guidelines/MedicalPhysics.aspx. Accessed August 8, 2007.
- Samei E, Lo JY, Yoshizumi TT, et al. Comparative scatter and dose performance of slot-scan and full-field digital chest radiography systems. Radiology. 2005; 235:940-949.
- Pisano ED, Yaffe MJ. Digital mammography.Radiology.2005;234:353-362.
- Reiner BI, Siegel EL, Hooper FJ, et al. Multi-institutional analysis of computed and direct radiography: Part I. Technologist productivity. Radiology.2005;236: 413-419.
- Reiner BI, Salkever D, Siegel EL, et al. Multi-institutional analysis of computed and direct radiography: Part II. Economic analysis. Radiology.2005;236:420-426.
Dr. Siegel is a Professor of Diagnostic Radiology and the Radiology Associate Vice Chairman for Informatics, Diagnostic Imaging, University of Maryland Medical Center; and the Director, Baltimore Veterans Affairs Medical Center, Baltimore, MD. He is also a member of the editorial board of this journal.
Digital radiography, despite its fascinating technology, its tremendous potential, and the fact that it remains the primary and dominant diagnostic imaging screening modality, is not considered by most radiologists to be "sexy." No major conferences for radiologists are currently dedicated to digital radiography, and the number of abstracts and papers about the technology is Lilliputian compared with those about multidetector computed tomography (CT), positron emission tomography/ CT, and magnetic resonance imaging (MRI). General radiography is arguably in danger of becoming a lost art. A thoracic radiologist trained before the use of picture archiving communications systems (PACS) recently lamented the fact that today's radiology residents seem to pay scant attention to "plain films"; they immediately ask for a CT study when shown a chest radiograph at the American Board of Radiology examinations in Louisville, KY. This seems unfortunate and ironic, not only because the roots of diagnostic radiology are in conventional radiography but also because this continues to be the most common type of imaging study performed in the United States and throughout the world. Conventional radiology continues to represent an important and fundamental diagnostic tool.
Digital radiography was originally conceived and marketed in the 1980s and early 1990s as a way to improve the image quality of film by taking advantage of an innovative detector (photostimulable plate) that had a substantially wider dynamic range than had been possible using film, performing image processing to extract the most clinically important imaging information, and printing this image to film. Initial versions of this system printed 2 images on a single film, each processed differently to present 2 perspectives of the radiograph.
By the early 1990s, however, it seemed increasingly clear that film's days were numbered. The substantial increases in computer workstation speed, network performance, and storage capacity (along with major reductions in prices) finally made it possible to create a practical filmless radiology department and healthcare enterprise. Conventional radiography was the one remaining nondigital modality in most departments, and digital radiography became an enabling technology not only for film-based image enhancement but for entirely filmless operation. Digital X-ray technology and options have expanded dramatically over the past several years (as has ambiguity about the terminology used to describe these options).
Current state-of-the-art digital X-ray systems offer excellent dynamic range with similar or reduced quantum detection efficiency (permitting dose reduction with equivalent image quality) and, along with image processing, offer significant potential to substantially improve on the image quality that is typically achieved with film. These systems combine the advantages of digital imaging, such as image processing, direct transfer to PACS, and elimination of film and associated chemicals, with the advantages of conventional X-ray, such as low cost, portability, flexibility in positioning, and very low radiation dose (typically <5% of the dose that is required for CT).
However, the overall quality of digital radiography studies in actual practice has not met the potential of the technology to improve on conventional film radiography and, in many cases, has actually fallen behind the quality achieved on film. This is the result of a combination of the increased complexity of digital radiography, limited training for technologists in digital X-ray techniques, relatively low interest by radiologists in the modality, and a general lack of understanding about digital image processing and the artifacts and pitfalls associated with it. 1,2 Despite initial reports by our group and others about decreased retake rates due to the improved dynamic range of digital radiography, image retake rates are still unacceptably high and image quality is too frequently suboptimal. 3
The mantra of PACS has been to have images available at any time and any place to anyone who needs to review them. The mantra of digital radiography must be to be able to acquire images any time and any place, make these images available immediately for quality review, and then send them to a PACS for near real-time display. This can be achieved either by having a cassette reader available in every hospital area in which studies are obtained or, alternatively, by using a technology that reads/processes the images immediately and then displays them for evaluation by the technologist and simultaneously sends them to the PACS. This type of immediate read/processing digital system has been introduced within the past few years and will continue to become faster, lighter, more rugged, and more affordable over time.
Portable digital radiography is currently the area with the greatest potential for improvement in digital radiography and will continue to be for the foreseeable future. Challenges for optimal or even adequate image quality include the following: portable generators with limited power; difficulties in positioning critically ill, unstable, or fragile patients for standard views; challenges with the use of grids in portable studies; limited time to obtain the study; and inability to visualize the image during the examination to determine whether the study was adequate. Another problem is the required trade-off between performing multiple studies and having to wait a relatively long time to process images, as well as the inefficiencies associated with taking plates down to the main department.
Additional clinical challenges include the need for improved productivity because of staff shortages and space limitations; the need to reduce costs to enable digital imaging solutions in lower-volume imaging locations, such as family practice, orthopedic, podiatric, and chiropractic settings; and the need to improve image quality and image acquisition and transmission times for remote locations (such as the intensive care unit, emergency department, and operating rooms), particularly in settings where patient positioning is challenging. Productivity can be enhanced by evaluating and redesigning workflow processes. Technologists should spend a higher percentage of their time performing studies than they do performing the clerical/manual steps typically required by many digital radiographic systems today. Multi-institution time/motion studies evaluated by our research team have documented that technologists spend >40% of their time on clerical and image quality assurance steps. Improving this imbalance will require integration of digital X-ray systems into the radiology and hospital information systems as well as additional automation of the technologists' workflow.
Despite major advances in digital radiography, the basic way in which images are obtained has not fundamentally changed during the past 100 years. In order to achieve a major advance in the evolution of digital radiography, we need to take advantage of the unique capabilities of digital X-ray technology, rather than using it as a mere substitute for film. For example, digital detectors have a different "k-edge" from that of film, giving us flexibility in rethinking the energy of the radiation in addition to dose. Digital systems make image subtraction relatively simple, and we can take advantage of this by utilizing dual-energy subtraction. We have made the transition to dual-energy subtraction for all outpatient examinations at our facility and have found that this improves sensitivity and specificity for lung nodules and other pathology as well as for bony lesions in the chest. We plan to perform research on this technology in other areas of the body. Dual-energy technology may give way to multienergy X-ray and photon-counting devices that, in the future, could provide even greater contrast and spatial resolution at reduced doses. Digital X-ray may bring a renaissance in the use of tomography and tomosynthesis because of the relative ease of computer processing of multiple images using technology developed for CT. This could produce much higher contrast images than are possible with planar X-ray techniques, with far lower doses than are achievable using CT. Digital image acquisition also facilitates the use of computer-aided detection of lung nodules, microcalcifications and masses on a mammogram, and detection of interstitial lung disease and life support lines in ways that would be difficult to achieve with film.
Conclusion
Despite the sometimes astonishing advances in CT, MRI, and optical and molecular imaging, the good news is that radiology's mainstay--conventional radiography--is making exciting and innovative advances. The challenge is to engage and educate the diagnostic imaging community about the very real advantages and potential that digital radiology is bringing to our most time-honored imaging modality.
REFERENCES
- Willis CE, Thommpson SK, Shepard SJ. Artifacts and misadventures in digital radiography.Appl Radiol.2004;33(1):11-20.
- Koenker RM. Digital radiography quality assurance: A technique for standardizing image appearance across CR and DR platforms. Appl Radiol. 2007;36(6):22-28.
- Siegel EL, Protopapas Z, Pickar E, et al. Analysis of retake rates using computed radiography in a filmless imaging department. Presented at the Radiological Society of North America Annual Meeting; December 3, 1996; Chicago, IL.