Digital radiography: The bottom line comparison of CR and DR technology
Images
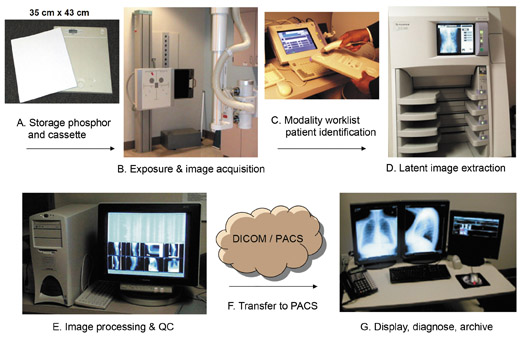
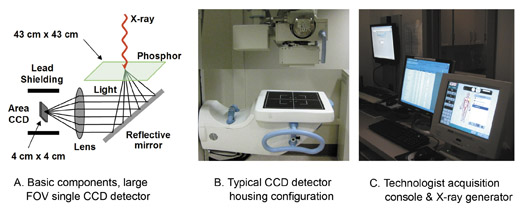
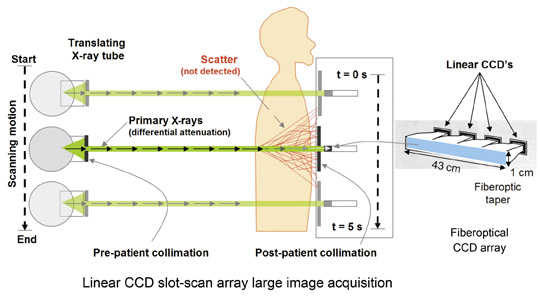
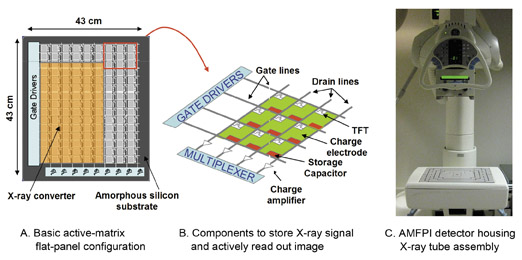
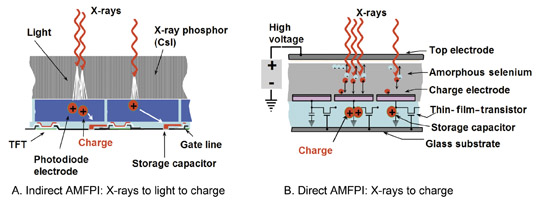
Dr. Seibert is a Professor and Assistant Chair of Informatics, Department of Radiology, University of California Davis Medical Center, Sacramento, CA.
Digital radiography detector systems were first implemented for medical applications in the mid 1980s, but the promise of digitalimaging was not realized until the early 1990s, in conjunction with the establishment of first generation picture archiving and communications systems (PACS). Atthe time, there was only one technology available to replace the analog screen-film detector-a cassette-based, passive acquisition photostimulable storage phosphor (PSP) and plate reader system, known as "computed radiography" (CR). This system closely emulated the screen-film paradigm. Alternate technologies for digital image acquisition appeared in the mid 1990s with the use of large field of view (FOV) X-ray phosphors and optical lens assemblies to focus the X-ray induced light output onto a small-area charge coupled device (CCD) photodetector array, as well as rectangular CCD arrays used with slot-scan geometries. Active-matrix flat-panel imager (AMFPI) systems appeared in the late 1990s and early 2000s, employing either an X-ray-to-light converter with photodiodearray, or a semiconductor material to directly convert incident X-rays into signals. Both CCD and flat-panel based detectors use an "active"readout of the image following acquisition to present the image immediately without further interaction by the technologist. Other technologies such as complementary metal-oxide semiconductor (CMOS) detectors were introduced in the same time frame as AMFPI's, but have not as of yet been successful, mainly due to problems with excessive electronic noise.
Beyond the digital detector characteristics are considerations for software for pre- and postprocessing of the digital image data, the user and modality interfaces, display monitors and calibrations. Many unique acquisition capabilities, such as dual-energy image tissue decomposition and limited-angle digital tomosynthesis, are important when considering future applications of specific importance.
The contents and discussion within this article are based on a "generic" description of PSP (CR) and DR detectors. Suffice it to say, there are a wide range and capability of detector systems within each "class" of digital detector technology, and many of the sweeping statements made may or may not be accurate with respect to a specific digital radiography device.
Digital radiography technology
Photostimulable storage phosphor (PSP) detectors
More commonly recognized as CR, the PSP detector "system" is comprised of 2 main components. The detector is usually a cassette-based storage phosphor that absorbs X-ray energy transmitted through the patient and temporarily stores the X-ray latent image as a 2-dimensional array of electrons trapped in semistable energy wells. The imaging plate "reader" is comprised of a scanning laser beam to stimulate the trapped electrons and produce "photostimulated luminescence" of a different wavelength that is optically separable from the stimulation wavelength. The reader also includes a light guide and photomultiplier assembly that extracts and processes the stored X-ray content to a sampling resolution on the order of 100 microns (0.1 µm), digital electronics to create the corresponding digital image, and an erasure stage to eliminate any residual signal and prepare for the next exposure. From the reader, all images proceed to a quality control workstation for image evaluation, annotation and transfer to PACS (Figure 1). Most often, the storage phosphor is layered on a flexible or solid substrate in a cassette enclosure, which allows for the ability to directly replace a screen-film cassette in a conventional radiography room. Thus there is the flexibility and portability of a cassette with digital radiography acquisition capability using existing X-ray equipment; this is CR's greatest asset. CR cassettes of various size and number, together with a high-speed imaging plate reader can service multiple X-ray rooms, resulting in a relatively low initial acquisition cost. However, the technologist must handle the cassettes and process the imaging plates in a manner similar to film, which can reduce patient throughput in a busy clinical room and increase labor costs, as the time to handle the exposed imaging plate to the reader and output of the X-ray image can often take several minutes (about 45 to 60 seconds to "read" the plate with the moving laser beam). Other expenses to consider are the need for high-frequency (e.g., 170 lines per inch) antiscatter grids for stationary (non-Bucky) applications such as portable bedside imaging and fixed grid cassette holders, and for readjusting phototimer sensitivity to account for the lower detection efficiency of the conventional PSP imaging plate compared to 400 speed screen-film detectors. Long-term costs include cassette and imaging platelongevity, maintenance and cleaning of the imaging plates and reader assembly, replacement of damaged detectors, and continuous oversight with a quality control program. 1
An alternate description of CR as "cassette radiography" in lieu of "computed" is a sign of the technological changes that are occurring-PSP technologies are now being implemented in enclosed housings, with high-speed parallel laser beam stimulation and photodiode array acquisition that fully reads the exposed storage phosphor in as little as 5 seconds, comparable to many "direct" DR detectors. Available a reportable flat-panel radiography detectors in a cassette form, some with wireless technology, which can provide active readout at the point of service without subsequent user interactions.
Technological developments of storage phosphors include compounds with less intrinsic lag during stimulation for faster readout times,"dual-side" phosphor deposition on a transparent substrate to improve X-ray detection and stimulated luminescence efficiency for a higher signal-to-noise ratio (SNR) with the same exposure, and "structured PSP" materials such as cesium bromide (CsBr) that simultaneously improve spatial resolution and detection efficiency. 2 Cassette-based CR detectors are available for digital mammography with special adjustments to the reader device and laser beam (e.g., 50 micron pixel sampling), and are a viable consideration for converting from screen-film particularly for operations that have a low patient volume and/or have relatively new mammography X-ray equipment. Initial capital outlay is certainly lower than a corresponding integrated digital radiography (or digital mammography) flat-panel detector system.
Charge-coupled device detectors
The design of a charge-coupled device (CCD)-based DR system is straightforward. The detector is comprised of a large FOV (e.g., 43 cm by 43 cm) scintillator that converts absorbed X-ray energy into light. It also includes an optical lens assembly to focus the light onto the photosensitive CCD array, and a CCD camera to integrate, scan and output the corresponding light image. While there were initially several configurations in early systems, today's CCD-based detector is typically comprised of a single-compound optical lens and a high-resolution CCD camera comprised of 9 million pixels (3000× 3000 pixels) to 16 million pixels (4000 × 4000 pixels). When referred back to the image plane, this results inimage pixel sizes of ~0.10 to ~0.14 µm (Figure 2). The photosensitive area of the CCD chip is actually quite small, on the order of 2.5 cm × 2.5cm to 4.0 cm × 4.0 cm, which is required to maintain extremely high charge-coupling efficiency and low-noise operation during the readout of the image. Thus, there is a large optical demagnification that is necessary to focus the full FOV light image onto the CCD sensor. One physical difficulty is the inefficiency of light collection caused by the dispersed light emission from the phosphor, resulting in only a small fraction that can be focused onto the CCD, thus potentially reducing the statistical integrity of information carried by the X-ray photons and increasing overall noise in the image. This is determined by the demagnification factor, conversion efficiency, luminance and directionality of the light emission. A non-structured phosphor such as gadolinium oxysulfide has a high light dispersion and corresponding low fraction of light that can be focused on the CCD, while a structured phosphor such as cesium iodide (CsI) produces a more forward-directed light output, so that the lens-light collection efficiency, and thus the SNR in the output image, is better for a given incident X-ray exposure. Newer, advanced CCD systems with a CsI phosphor have proven to be reasonably efficient, particularly when using higher kilovolt peak (kVp) techniques that produce more light photons per absorbed X-ray photon. One minor disadvantage in some positioning situations is the relatively large and bulky enclosure of a CCD-based DR system, necessitated by placing the CCD out of the direct X-ray beam and using mirror optics to reflect the light to the photosensor array.
Linear CCD arrays optically coupled to a scintillator by fiberoptic channel plates (often with a demagnification taper of 2:1 to 3:1) are used in slot-scan geometries (Figure 3). A significant advantage is pre- and postpatient collimation that limits X-ray scatter and allows grid-free operation with equivalent image quality (in terms of SNR) of a large area FOV at 2 to 4 times less patient dose. Disadvantages include the extended exposure time required for image acquisition with potential motion artifacts and reduced X-ray tube efficiency. Nevertheless, imaging systems based on slot-scan acquisition have provided excellent clinical results for dedicated chest and full-body trauma imaging.
Complementary metal-oxide semiconductor (CMOS) detectors
CMOS light-sensitive arrays are based upon a crystalline silicon matrix and are essentially random access memory "chips" with built-in photodiodes, storage capacitors, and active readout electronics, operating at low voltage (3 to 5 volts) for image acquisition and readout. The ability to randomly address any detector element on the chip enables opportunities for automatic exposure control (AEC) capabilities that are not easily performed with a CCD photo detector. However, electronic noise has been a problem that has slowed the introduction of this technology. Construction of a large-area detector is also a hurdle for detector arrays directly coupled to the X-ray converter, due to the maximum detector element size currently achievable (on the order of 50 µm). CMOS detector technology applications for radiography are currently unavailable, but recent development of a CMOS-based digital mammography detector compatible with conventional mammography systems has beenintroduced for small FOV (10 cm × 10 cm) application. 3
Active-matrix, flat-panel imagers
AMFPI technologies are based on thin-film-transistor (TFT) arrays, made from amorphous silicon, upon which lithographic etching and material evaporation on the micron scale produces the electronic components and connections necessary for detector operation. The flat-panel substrate is divided into individual detector element (del) compartments, arranged in a row and column matrix, typically with a spacing dimension of 70 microns to 250 microns, depending on the detector specifications. Components within each del include a thin-film-transistor (essentially an electronic switch), a charge collection electrode and a storage capacitor. Electronic interconnections including gate lines (rows) and drain lines (columns) are connected to each of the TFTs to control the on/off status of each of the dels and to provide the conduction paths to the charge amplifiers, respectively. All of the TFTs are closed during an exposure to collect X-ray-induced charge proportional to the incident X-ray fluence in each del. After the exposure, active readout of the array occurs one row at a time by activating the respective gate line, which turns on the TFTs and allows the stored charge to flow along the columns from each del capacitor via drain lines to the corresponding charge amplifier. Banks of amplifiers simultaneously amplify the charge, convert to a proportional voltage, digitize signals in parallel from each row of the detector matrix and produce a corresponding row of integer values in the digital image matrix (Figure 4). This process is repeated for each row in the matrix. Detector readout speed is governed by the intrinsic lag characteristics of the X-ray converter material,switching speed of the TFT array electronics and the number of independent charge amplifier arrays that can function in parallel.
Limits to spatial resolution for TFT arrays include fill factor, electronic interconnections and manufacturing yield. Fill-factor is a term describing the active charge collection area to the total area of the del, 4 and ideally is 100% for most efficient collection of X-ray information. However, because electronic components and connection lines occupy space on the substrate, with conventional TFT designs the fill factor is less than ideal, this is more severe for smaller del areas of ~0.1 µm (e.g., <50%), and can ultimately limit the minimum size of the del (highest spatial resolution achievable). To increase the spatial resolution by a factor of 2 requires a 4 times increase in the number of electronic interconnections and a much greater complexity in the design of the detector. The probability of malfunctioning dels, gates and drains increases with higher spatial resolution requirements, which can drastically lower the yield. While there is no "perfect" TFT, small defects are corrected by mapping bad dels and using interpolation methods of nearby elements to "fill-in" the expected response. This is successful as long as the interpolation does not exceed more than a couple elements in the local area that require repair. In addition, flat-field preprocessing methods (beyond the scope of the topic presented here) are common and required for all digital radiography detectors (DR and CR) to compensatefor variations in detector gain and response across the detector. 5 The frequency of flat-field calibration suggested by manufacturers (weekly, monthly or annually) is dependent on the detector and the propensity for going out of calibration.
AMFPIs are classified into "indirect" and "direct" X-ray converters, which are based on the physics of X-ray charge conversion (Figure 5). Indirect AMFPIs use an X-ray phosphor material layered on a TFT-photodiode array substrate, where light photons are the intermediary between X-ray absorption and charge generation on the TFT array detector element. Structured CsI is currently the most widely used phosphor, mainly for excellent X-ray detection efficiency and good spatial resolution capabilities. Incident X-rays are absorbed, proportional light intensity is produced, proportional charge amplitude is generated by the photodiode upon exposure to the light, and the resultant charge (X-ray signal) is stored at the local capacitor. Several additional lithography steps are needed for including the photodiode components during the manufacturing process of the TFT array compared to TFT detectors without photodiodes. 6 Manufacturers also create arrays in two different ways: monolithic, whereby the whole detector is created from one substrate material, and smaller subdetector arrays that are joined together as quadrants to create the full FOV detector. Both manufacturing methods produce excellent detectors, but there is a limit on the size of the monolithic production that is slightly smaller than the conventional 43 cm x 43 cm large FOV. On the other hand,the process of joining four independent detector subarrays into one large array can have meshing defects, and while not visible on inspection of the image, certainly have a noticeable impact on the noise characteristics of the detector as determined by sophisticated noise-power spectrum analyses. 7
Direct AMFPIs use a semiconductor X-ray converter that directly produces electron-hole pairs in proportion to the incident X-ray intensity. Amorphous selenium (a-Se) is currently the semiconductor of choice, and is layered between two electrodes connected to the bias voltage and a dielectric layer. The ion pairs are collected under a high voltage (10 to 50 volts per micron of thickness) in order to prevent recombination,and to prevent lateral spreading of the information carriers within the a-Se material. A simple TFT-array structure is used to collect the resultant charge, and excellent spatial resolution is achieved, chiefly limited by the size of the del. Fill-factor penalties are less of an issue with directAMFPI devices, as electrode designs can funnel charges along electric field lines. However, charge trapping and residual charge lag can occur within the semiconductor layer, which can be quite problematic for image ghosting from previous exposures. Detector "relaxation" after an exposure is often performed to release trapped charges within the substrate. Additionally, if the a-Se substrate beginsto crystallize, the semiconducting properties of the converter are reduced and the detector must be replaced. Improved manufacturing methods have reduced trapping and lag to low levels, and the propensity for crystallization is minimized by placing a small amount of arsenic doping to the semiconductor layer during manufacturing.
Which is better: indirect or direct AMFPI detectors? There are no clear answers to this often-asked question; both technologies have advantages and disadvantages. With current technological implementations, in general, it appears that indirect AMFPIs have fared better with conventional radiography applications and with high-speed image acquisition and readout (e.g., dual-energy radiography and digital tomosynthesis). Direct AMFPIs have performed better in high-resolution environments such as digital mammography. Of course, as technology changes, so do the answers.
When deciding on an AMFPI detector technology for clinical implementation, important considerations are the warranty and guarantees for detector longevity and uptime, as replacement of the detector electronics can be a very expensive proposition.
Comparing passive PSP (CR) and active DR technologies
Advantages for cassette-based PSP (CR)
CR represents the digital technology that can directly replace screen-film imaging at a low initial investment cost and still use existing X-ray equipment with minimal changes or calibration requirements. Multiple size cassettes allow the proper detector size to be used for various procedures, and provide the ultimate in positioning flexibility for any examination. New "point of service" imaging plate readers onboard with portable X-ray systems can provide rapid turnaround beside radiography examinations and verification of positioning before leaving the patient area. Smaller and highly capable in-room dedicated single-plate readers can achieve good throughput by allowing the technologist to acquire the next image in a series while processing the previously exposed imaging plate. Capital outlay required to introduce digital radiography into the clinical environment is a clear advantage of CR systems over most DR technologies, due in large part to the ability to use existing X-ray infrastructure. While proponents of DR technology point towards the lower detection efficiency of standard PSPimaging plates and required higher dose to achieve a given SNR, introduction of dual-side readout phosphors and CsBr structured storage phosphors have resulted in a significant increase in the detection efficiency on par with some DR-based detectors.
Bottom line: Flexibility, convenience, ease of implementation and initial (low) costs are the hallmarks of cassette-based CR detectors.
Advantages for active, integrated DR detectors
Integrated DR detector systems have a great advantage with technologist workflow and patient throughput, particularly for ambulatory outpatient imaging systems. This is chiefly due to the rapid display of the acquired image seconds after the exposure. A specific DR system for dual-energy chest imaging at UC Davis provides a patient turnaround for a 2-view chest in under 2 minutes from patient positioning to image acquisition to review/verification of the images and sending to the PACS. A corresponding room using CR cassettes easily takes 5 to 7 minutes or more, much of the time is spent handling cassettes and waiting for the image readout and processing. AMFPIs typically have a significantly higher detective quantum efficiency compared to a conventional PSP/CR cassette (e.g., ~60% compared to ~30%), meaning that reduced X-ray techniques with lower patient doses can be used for similar image quality and SNR.
Recent entry of wired, and now wireless, portable DR detectors are treading on the domain of PSP cassettes in many instances, making a strong case for the possible elimination of PSP detectors in the future, but there is still a way to go before that will happen. Certainly the retrofit market for implementation of add-on DR detectors is poised for rapid growth as users want the benefits of DR workflow without the huge capital investment of a new, integrated DR system. Because of high acquisition speed, advanced acquisition technologies, such as dual-energy radiography and digital tomosynthesis, are possible in situations that can benefit from these current, and future, cutting-edge capabilities.
Bottom line: Image acquisition speed, advanced acquisition technologies and excellent image quality are the hallmarks of DR systems.
Disadvantages for cassette-based PSP (CR) detectors
The delay between exposure and readout with labor-intensive handling is a major disadvantage of cassette-based PSP detectors, particularly in situations that require the technologist to be away from the patient. Readout and processing time of imaging plates can be long, and for single plate readers, overexposures can take a long time to "erase" the residual signals before another imaging plate can be inserted. PSP detectors are always "on," meaning that they are prone to background radiation and scattered radiation if improperly stored next to, or in, a radiographic room. It is very important to implement an erasure of imaging plates that have not been used frequently, particularly after a long weekend as a precaution to eliminate any potential contrast degrading background radiation signals on patient images. Multiple PSP detectors, while a nice "redundancy" feature, can also be a chore in terms of inventory, cleaning, quality control testing and general maintenance.
Disadvantages for active, integrated DR detectors
Positioning flexibility (e.g., cross-table lateral acquisitions, exotic views) is often difficult or impossible to achieve with many DR systems (although newer integrated designs and portable DR detectors are overcoming some of the early system design limitations). Initial capital costs are extremely high (on the order of 3 to 5 times the cost of a high-quality CR reader and imaging plates), making the case for return on investment crucial if the workload can't justify the throughput advantages. Redundancy concerns and the cost of detector replacement are serious issues, and any down time in a busy, high-throughput room can be detrimental to operating costs and timely delivery of patient care.
Best of both: Combining cassette-based CR and integrated DR
In most major hospitals, there will always be the need for flexibility and for efficient throughput for radiography, and with the current status of both technologies, they nicely coexist. Integration of CR and DR in a single room with similar image processing and concatenation of studies under a single accession number is an attribute that allows for maximum productivity under one acquisition console, which has been successfully implemented in an outpatient clinic in the UC Davis Health System. In low-volume situations that do not require high throughput for radiography examinations, the implementation of cassette-based CR has been the choice for replacement of screen-film technology. However, with advancements and lower costs likely, the potential of using a robust, low-cost portable DR detector in lieu of CR is very possible in the coming years.
Replacement strategies for historically cassette-based CR systems at the UC Davis Health System are focused on implementation of AMFPIindirect detectors in targeted service areas, side by side with radiographic rooms that continue to use cassette-based CR. This has proven to be an effective way of providing the best of both technologies by taking advantage of the benefits of each. While the investment in CR technology remains strong, there is no doubt that advanced imaging capabilities such as dual-energy radiography and digital tomosynthesis volume imaging have resulted in a focus on and greater emphasis of flat-panel DR technologies for targeted procedures that can reduce the need for computed tomography, MRI and ultrasound examinations.
The American College of Radiology has recently published practice guidelines for digital radiography 8,9 including a review of digital radiography technologies, clinical considerations for implementation and quality control recommendations. This information is an excellent resource for all individuals responsible for digital radiography in the clinical environment.
Conclusion
Digital radiography detectors (CR and DR detector systems) are now in the majority. Cassette-based photostimulable storage phosphor detectors comprise the largest segment of digital detectors; however, cassetteless, integrated detectors are increasing in areas that demand high efficiency and high throughput. The historical comparison of "CR vs. DR" while still a consideration for making an informed decision for choice of adigital radiography system, is of less importance, as the advance of technology has blurred the differences. Image acquisition speed of AMFPIs is enabling advanced acquisition and processing capabilities such as dual-energy radiography and digital tomosynthesis, which will likely become common methods that are used to overcome the superimposition limitations of conventional projection radiography and result in more accurat ediagnoses. Just expect to pay more for advanced technology capabilities, and be sure to justify the expense in terms of realistic workloads and throughput requirements; otherwise do not expect a decent return on investment. Knowledge of DR system characteristics, advantages, disadvantages and operational details provide the insight and confidence to make a reasonable, informed decision on equipment selection and optimization of digital radiography implementation for a specific purpose.
REFERENCES
- Seibert JA, Bogucki TM, Ciona T, et.al. Acceptance testing and quality control of photostimulable storage phosphor imaging systems: Report of AAPM Task Group 10. AAPM Report #93. American Association of Physicists in Medicine, College Park, MD. (2006) Available at http://aapm.org/pubs/reports/ RPT_93.pdf. Accessed April 27, 2009.
- Leblans PJ, Struye L, Willems P. New needle-crystalline CR detector. Proc SPIE. 2001;4320:59-67.
- Baysal MA, Toker E. CMOS Cassette for digital upgrade of film based mammography systems. Proc SPIE. 2006;6142:61421Q.
- Pisano ED, Yaffe MJ. Digital mammography. Radiology. 2005;234:353-362.
- Seibert JA. Digital radiographic image presentation: Pre-processing methods. In: Samei E, Flynn MJ, eds. 2003 Syllabus: categorical course in diagnostic radiology physics - Advances in digital radiography. Oak Brook, IL: Radiological Society of North America (RSNA);2003:147-151.
- Rowlands JA, Yorkston J. Flat panel detectors for digital radiography. In Medical Imaging, Volume 1, Physics and Psychophysics, Beutel J, Kundel HL, Van Metter RL eds. Societyof Photooptical and Instrumentation Engineers (SPIE). Bellingham, WA. 223-328, 2000.
- Samei E, Saunders RS, Lo JY, et al. Fundamental imaging characteristics of a slot-scan digital chest radiographic system. Med Phys. 2004;31:2687-2698.
- Williams MB, Krupinski EA, Strauss KJ, et al. Digital radiography image quality: image acquisition. JACR. 2007;4:371-388.
- Krupinski EA, Williams MB, Andriole K, et al. Digital radiography image quality: Image processing and display. JACR. 2007;4:389-400.